Introduction
Meat including pork, chicken, beef comes from skeletal muscle of farm animals. Although humans consume meats as a kind of food, skeletal muscles are a specialized tissue to convert chemical energy, such as adenosine triphosphate (ATP), to mechanical energy, locomotion, via muscle contraction (Aberle et al., 2001). Adenosine triphosphates are required during contraction and relaxation of skeletal muscle. Thus, skeletal muscles continuously metabolize and synthesize the nutrition to get ATPs (Aberle et al., 2001; Warriss, 2010).
Patterns of ATP synthesis and metabolism in skeletal muscles are influenced by muscle fiber characteristics (Ryu and Kim, 2005; Choe et al., 2008, 2009; Choe and Kim 2014). Skeletal muscles
of animals are composed of different types of fibers, usually categorized as fiber type I, IIA, and IIB. Each muscle fiber type has different biochemical, physiological, and biophysical characteristics such as contraction speed, oxidative or glycolytic metabolic traits, metabolites contents (Schiaffino and Reggiani, 1996; Klont et al., 1998; Karlsson et al., 1999; Park et al., 2017). So, fiber type composition of muscle can strongly affect not only antemortem muscle metabolism but also postmortem conversion of muscle to meat (Ryu and Kim, 2005; Choe et al., 2008, 2009; Choe and Kim, 2014). Therefore, this paper was to overview muscle metabolism, muscle fiber characteristics, and their influence on meat quality.
Muscle metabolism and metabolites and their relationship with meat quality
Muscle metabolism
Living and postmortem muscles maintain a stable and constant condition as possible (homeostasis) against dramatic changes. Adenosine triphosphate is also maintained at a constant level due to homeostasis. In living muscles, energy (ATP) production has two main alternative routes; the oxidative (aerobic) and the glycolytic (anaerobic) pathways (Pösö and Puolanne, 2005). The oxidative pathway is the most efficient way because it generates approximately 36 molecules of ATP via the complete aerobic oxidation of glucose-6-phosphate. However, this pathway requires a sufficient amount of oxygen and thus is adequate when muscles are working slowly (Yla-Ajos, 2006). On the other hand, the glycolytic pathway occurs when the amount of oxygen is insufficient or muscle are working rapidly. This glycolytic pathway changes glycogen, the polymer of glucose and the major energy source reserved in muscle and liver, into lactate generating 3 molecules of ATP. In living muscles, the lactate formed by the glycolytic pathway is converted back to glucose and glycogen in the liver through the circulation system (Pösö and Puolanne, 2005; Choe et al., 2008).
After slaughter/exsanguination, blood is removed and circulation system failed, resulting in no more oxygen supply to muscles. The muscles lack oxygen and becomes an anaerobic condition, so oxidative metabolism does not work. Initially, ATP is buffered by the creatine kinase- and myokinase-catalyzed reaction (Scheffler and Gerrard, 2007). Phosphocreatine (PCr) is present at a higher concentration (18 - 19 µmole/g) than ATP (6.6 - 6.8 µmole/g) in porcine longissimus muscle (Bendall, 1973). The enzyme creatine kinase catalyzes rephosphorylation of adenosine diphosphate (ADP) to ATP using PCr (1). Additionally, myokinase catalyzes the conversion of two ADP to adenosine monophosphate (AMP) and ATP (2) (Scheffler and Gerrard, 2007).
PCr + ADP → ATP + creatine (1)
2 ADP → ATP + AMP (2)
These two reactions allow the muscle to quickly increase ATP production in order to keep cellular ATP constant level and maintain homeostasis. However, when about 70% of the initial PCr content has been degraded, ATP levels rapidly decline (Bendall, 1951; Scheffler and Gerrard, 2007), and muscle glycogen must be metabolized by glycolysis in order to rephosphorylate ADP to ATP (Scheffler and Gerrard, 2007). Glycolysis produces waste products, such as lactate, a hydrogen ion (H+), and heat. Because postmortem muscle does not have the means to remove waste products, lactate and H+ accumulates in muscle tissue, and it causes a decline of muscle pH (Scheffler and Gerrard, 2007). When ATP breakdown exceeds ATP synthesis by glycolysis, less the amount of ATP is available to break actomyosin and thus the formation of actomyosin decreases sarcomere length, resulting in the onset of rigor mortis. Rigor mortis is completed when the ATP supply is exhausted (Scheffler and Gerrard, 2007).
Muscle metabolites and meat quality
Postmortem muscles generate ATP via enzyme reactions using PCr and the glycolytic pathway. Especially, the glycolytic pathway converts glycogen reserved in muscle into lactate as an end-product. Lactate formed by glycolytic pathway accumulated in the muscle, resulting in decline of muscle pH. Thus, glycogen and lactate in muscle are important metabolites when discussing the conversion of muscle to meat at postmortem.
The rate and extent of metabolism and pH decline during the conversion of muscle to meat significantly impact the development of fresh meat quality attributes (Scheffler and Gerrard, 2007). Normally, pH declines gradually from 7.4 in living muscle to roughly 5.6 - 5.7 within 6 - 8 h of postmortem and then has an ultimate pH at 24 h (pH24 h) of about 5.3 - 5.7 (Briskey and Wismer-Pedersen, 1961). However, muscles with a rapid glycolysis exhibit an accelerated decline in muscle pH and produce large amounts of heat, which slows carcass chilling. In particular, muscle pH declines rapidly more less than 6.0 during the first hour after slaughter and reaches an ultimate pH of 5.3 - 5.7. The onset of rigor mortis at high temperature and low pH causes the excessive denaturation of approximately 20% of the sarcoplasmic and myofibrillar proteins (Honikel and Kim, 1986; Scheffler and Gerrard, 2007). Excessive denaturation of sarcoplasmic proteins results in greater precipitation and it is largely responsible for paler pork color, while excessive denaturation of myofibrillar proteins accounts for the reduced water holding capacity (WHC) in pale, soft, and exudative (PSE) pork (Joo et al., 1999).
Initial glycogen content in the muscle at slaughter is the main factor for extent of postmortem glycolysis. Postmortem glycolysis uses glycogen as a main substrate (Pösö and Puolanne, 2005; Yla-Ajos, 2006; Scheffler and Gerrard, 2007). Glycogen is degraded into glucose subunit (glycogenolysis) and is left with one less glucose molecule by glycogen phosphorylase (Yla-Ajos, 2006). The free glucose is in the form of glucose-1-phosphate and is converted into glucose- 6-phosphate to be used for metabolism by phosphoglucomutase (Yla-Ajos, 2006; Scheffler and Gerrard, 2007). Glycolysis uses glycogen or glucose and produces ATP and lactate (Pösö and Puolanne, 2005; Yla-Ajos, 2006; Scheffler and Gerrard, 2007). An extended pH decline at normal rate due to high initial glycogen content in the muscle at slaughter contributes to a low ultimate pH (roughly 5.3 - 5.5), resulting in “acid meat” (Scheffler and Gerrard, 2007). The reduced net charge of myofibrillar proteins owing to abnormally low pH attracts thick and thin filaments closer together and it forces water out of the myofilament lattice (Irving et al., 1989; Offer et al., 1989). In addition, Joo et al. (1999) have reported that a decreasing ultimate pH declines solubility in sarcoplasmic protein, which contributes to paler pork color. Thus, the rate and extent of decline in muscle pH at the postmortem significantly influence on ultimate meat quality and muscle metabolites, such as glycogen and lactate. Choe et al. (2008) and Choe and Kim (2014) reported the relationships between muscle glycogen and lactate measured at 45 min and 24 h postmortem muscle, glycolytic rate, and pork quality. In those studies, pork with lower glycogen and higher lactate contents at 45 min postmortem exhibited rapid glycolytic rate. Muscles with high glycogen and lactate contents at 45 min postmortem showed rapid postmortem glycolysis, severe denaturation of myofibrillar and sarcoplasmic proteins, and thus deteriorative pork quality like PSE condition. These results indicate that muscle metabolites, such as glycogen and lactate, could explain variations in the postmortem glycolytic rate, protein denaturation, and ultimate meat quality.
Muscle fiber characteristics and their relationship with meat quality
Muscle fiber characteristics
Meat is a result of postmortem changes of skeletal muscle. Skeletal muscle is composed of different types of fibers. Each fiber type has different metabolic and contractile characteristics, such as oxidative and glycolytic capacities, contractile speed, color, content of mitochondria, myoglobin, glycogen, lipid, capillary density, resistance to fatigue, and fiber size (Schiaffino and Reggiani, 1996; Klont et al., 1998; Karlsson et al., 1999) (Table 1). The most widely accepted classification is based on differences in the acid and alkaline stability of the myofibrillar ATPase reaction (Brook and Kaiser, 1970; Ryu and Kim, 2005) (Fig. 1). According to this method, after acid or alkaline pre-incubations, muscle fiber types are divided into three types, I, IIA, and IIB. Muscle fiber type I, also known as red muscle fiber, is small and slow contracting. It has high contents of mitochondria, myoglobin, lipid, high capillary density, low content of glycogen, and strong resistant to fatigue. Therefore, muscle fiber type I have a predominantly oxidative metabolic capacity (Brook and Kaiser, 1970; Ryu and Kim, 2005). On the other hand, fiber type IIB, also known as white muscle fiber, is large, and fast contracting. It has low contents of mitochondria, myoglobin, low capillary density, and weakly resistant to fatigue. But glycogen content of type IIB is high, so it has a glycolytic and only weakly oxidative metabolic capacity (Peter et al., 1972; Hintz et al., 1984; Karlsson et al., 1999). The intermediate characteristic between type I and IIB is seen in muscle fiber type IIA. The comprehensive characteristic of a given muscle, especially metabolic characteristic, is determined by its relative composition of different muscle fiber type (Highley et al., 1999; Morita et al., 2000; Ryu et al., 2002). Therefore, it is generally accepted that the different fiber type composition of muscles may have different effects on postmortem changes during conversion of muscle to meat. Consequently, it determines the ultimate meat quality (Karlsson et al., 1999; Brocks et al., 2000; Ryu and Kim, 2006).
Fig. 1.
Representative image of porcine longissimus dorsi muscle stained using mATPase activity after pre-incubation at pH 4.7. Abbreviations: I, muscle fiber type I (slow-twitch & oxidative); IIA, muscle fiber type IIA (fast-twitch & oxido-glycolytic); IIB, muscle fiber type IIB (fast-twitch & glycolytic).
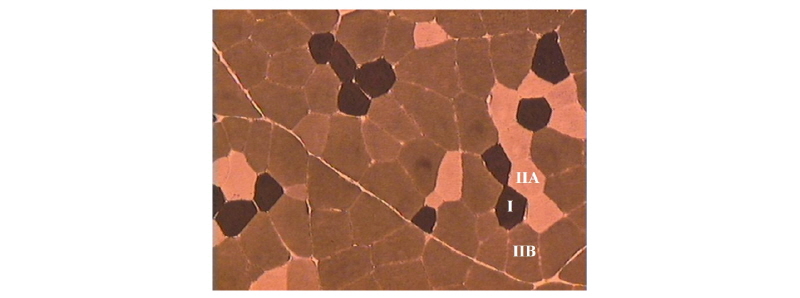
Muscle fiber characteristics and meat quality
Muscle fiber characteristics, such as muscle fiber number, size, and composition, are one of the factors that strongly affect the rate of postmortem metabolism, pH decline, and ultimate meat quality (Ryu and Kim, 2005). The total fiber number has been related to muscle mass, growth rate, and meat quality (Vann et al., 1998; Highley et al., 1999; Gentry et al., 2002). It is generally accepted that the low muscle fiber number correlated with greater fiber hypertrophy, and this may be associated with stress susceptibility and poor meat quality in modern meat-type breeds (Fiedler et al., 1999). The study of Lengerken et al. (1997) demonstrate the relationship between muscle fiber number and pH value in pork, and meat percentage at slaughter. They also investigated the optimum range of muscle fiber number for the high meat percentage and good meat quality at a moderate fiber size.
Several studies indicated that the size of the fibers may be of importance for muscle quantity (Staun, 1963; Fiedler et al., 2003), and meat quality (Allen et al., 1966; Fiedler et al., 2003). In modern meat type pigs, larger muscle fiber tends to have lower numbers of mitochondria (Rasmussen et al., 1996) and low capillary density (Fiedler et al., 1993), and they are similar characteristics with fiber type IIB (Rasmussen et al., 1996). Giant fibers were often observed in muscle presenting PSE meat properties (Solomon et al., 1990).
There are studies for the relation between muscle fiber composition and meat quality. In pigs, the occurrence of the PSE condition was generally related to content of fiber type IIA, and IIB (Henckel et al., 1997; Klont et al., 1998; Candek-Potokar et al., 1999). According to Fiedler et al. (1993, 1999), higher percentage of fiber type IIB correlated with PSE meat condition and with stress susceptibility. Pigs with a higher proportion of fiber type IIB showed more rapid decline of pH, lower WHC, and lighter color than pigs with the lowest proportion of the fiber type IIB (Carpenter et al., 1963; Henckel et al., 1997; Klont et al., 1998; Candek-Potokar et al., 1999). A similar result was also observed in the study of Ryu and Kim (2005). In that study, area and number composition of muscle fiber type I and IIB were significantly correlated with the postmortem metabolic rate and ultimate pork quality. Another study showed that content of slow or fast myosin heavy chain (MHC) isoforms, which determine muscle fiber types, influence on muscle metabolites contents, postmortem glycolytic rate, and subsequently ultimate pork quality like muscle fiber composition. Similar results can be found in other published papers (Choe et al., 2008; Choe and Kim, 2014), indicating that higher composition of fiber type IIB was associated with rapid and extended decline of muscle pH and finally deteriorative pork quality. Furthermore, Karlsson et al. (1999) have reported that composition of muscle fiber type determines the metabolic characteristic of muscle, and it could influence energy metabolism in live animals. Thus, contents of circulating metabolites, such as glucose and lactate, could be variable by muscle fiber type composition. It has also potential to influence on metabolic characteristic of muscles when animals are subjected to stressful conditions, such as adrenaline-induced glycogenolysis.