Introduction
An excess level of salts in the soil or water is the major factor limiting plant productivity in many areas of the world. Exposure to high salinity results in osmotic stress, which leads to an inhibition of photosynthesis and cell growth and increased production of reactive oxygen species (ROS) (Chaves et al., 2009; Miller et al., 2010). Many plants become acclimated to high salinity by mediating ion homeostasis, osmolyte biosynthesis, water uptake and transport, toxic radical scavenging, and coordination of long-distance responses (Hasegawa et al., 2000). These adaptive responses can also involve changes in levels of amino acids and organic acids, regulation of carbohydrate and polyol metabolism, and lipid composition (Wu et al., 1998; Sanchez et al., 2008).
High concentrations of proline, glycine betaine, soluble sugars, fructans, and polyols are accumulated in salt-stressed plants (Parida and Das, 2005). In leaves of Morus alba, the amounts of free amino acids and soluble proteins rise under low salinity but decline under high salinity (Agastian et al., 2000). Yields of essential oils and fatty acid compositions in the leaves of coriander (Coriandrum sativum) are differently affected by salt stress, and their levels of polyunsaturated fatty acids, which function as major nutrients, are reduced as the degree of salinity increases (Neffati and Marzouk, 2008). In soybean (Glycine max) plants, salt stress elevates the amounts of sodium and chloride while diminishing those of potassium, calcium, and magnesium (Essa, 2002). These compositional changes under salinity play important roles in protecting cell membranes, detoxifying ROS, and adjusting the osmotic balance (Parida and Das, 2005). However, previous research of this topic has tended to focus on leaves or roots rather than other edible portions of a plant, including the grain.
As a cereal grain, rice (Oryza sativa L.) is the most widely consumed staple food. High salinity is a major source of abiotic stress for that crop, particularly during the seedling stage, and it greatly reduces yields in vast areas worldwide (Kumar et al., 2013). Verma and Neue (1984) have reported that soil salinity influences the mineral composition of rice shoots, straw, and grain. However, changes in the concentration of an individual mineral vary according to organ type, with concentrations of nitrogen, magnesium, and copper increasing in the shoots and straw but remaining unchanged in the grains. De Pascale et al. (2005) have suggested that salinization-related accumulations of sodium and chloride in Brassica oleracea are lower in the heads than in the leaves, indicating that the greater accumulation of toxic ions in the leaves may help preserve the integrity of reproductive organs such as the inflorescence. Nevertheless, few studies have examined potential compositional changes in grains that are exposed to actual salt stress conditions (Thitisaksakul et al., 2015).
Compositional analysis of rice grains is of great importance when evaluating quality and nutritional value. We previously described compositional differences associated with drought-tolerant transgenic rice that over-expresses AtCYP78A7 in response to drought (Nam et al., 2014; Nam et al., 2015, 2016). In our current research, we investigated the metabolic and nutritional profiles of rice during the imposition of NaCl stress. Plants were cultivated under either salt or non-salt stress conditions. Metabolic variations were monitored in shoot and root samples collected at various growth stages as well as in grain samples. In addition, levels of proximates, amino acids, fatty acids, minerals, vitamins, and anti-nutrients were evaluated in the grain.
Materials and Methods
Plant material and growing conditions
Seeds of ‘Hwayoung’ rice (Oryza sativa L.) were sterilized with prochloraz solutions and sown in a seedbed filled with commercial potting soil developed for rice (Chalgama; Chamgrow Co., Hongseong, Korea). They were then cultured on a greenhouse bench at 28 ± 3°C / 22 ± 3°C (day/night) and under long-day (16 - h) conditions. Five weeks after sowing, seedlings were transplanted into 24 rubber pots (770 cm diameter × 480 cm height; 16 seedlings per pot) that each contained 160 L of the commercial potting soil. The pots were placed under a rainout shelter established in an experimental field at the Korea Research Institute of Bioscience and Biotechnology, Cheongju, Korea (36º 43´ N, 127º 26´ E; elevation, 35 m). After each pot was filled with tap water, that level was maintained with an automatic irrigation system.
Salt stress treatments
Salt stress was induced at four weeks after transplanting. Because soils are classified as saline when the electrical conductivity is 4 dS/m or higher (equivalent to 40 mM NaCl; Munns and Tester 2008), we subjected plants to salt stress by adding approximately 50 mM NaCl to the soil as mentioned in our previous study (Nam et al., 2017). Of the 24 pots, 12 were treated with 40 L of solutions containing 0.3% sodium chloride (NaCl, 51 mM) while the other 12 received only water. Irrigation was stopped during the first two weeks of this stress treatment. Thereafter, plants were re-supplied with tap water to recover for seven weeks until a second round of salt stress was imposed as described above. At the stages of tillering (2 weeks after first salt stress), heading (8 weeks after first salt stress), and ripening (14 weeks after first salt stress), one seedling from each pot was randomly sampled. Heights were recorded along with shoot fresh weights (FWs) and dry weights (DWs) and root FWs. The height of each plant was measured from ground level to the longest tip of the leaf blade. Shoot dry weights were determined after the tissues were oven-dried at 65°C for 5 d.
For the integrative analysis of metabolites, six pots were randomly selected from the 12 replicate pots and one seedling was randomly harvested from each pot at the stages of tillering, heading, and ripening. Shoots and roots were sampled at all three stages. Grains were collected at the ripening stage, then air-dried and hulled to obtain brown rice. All samples were freeze-dried (FreeZone 2.5 Freeze Dry System; Labconco, Kansas City, MO, USA), ground into fine powder, and immediately stored at – 80°C.
For the compositional analysis of grains, we randomly selected four pots from each treatment group of 12 and harvested the grains, then pooled them to obtain a composite sample. For the comparison of salt-treated and non-treated plants, the composition of three biological replicates was analyzed. After harvesting, whole grains were dried to a final moisture content of 11 to 14% and hulled to obtain brown rice. The dried samples were then ground into fine powder for analysis.
Extraction of metabolites
Metabolites were extracted for gas chromatography–mass spectrometry (GC–MS) analysis according to the method of Lisec et al. (2006), with some modifications. Briefly, 100 mg of frozen powdered sample was extracted with 1.4 mL of methanol and 60 μL of ribitol at 70°C for 10 min. The extracts were centrifuged at 11,000 g for 10 min and the resulting supernatant was combined with 750 μL of chloroform and 1.4 mL of water. After centrifugation at 2,200 g for 15 min, 150 μL of the upper aqueous layer was completely dried with a centrifugal vacuum concentrator (Genevac, Ipswich, UK). Two steps of derivatization were performed on the extracted hydrophilic metabolites. First, 40 μL of methoxyamine hydrochloride in anhydrous pyridine (20 mg mL-1) was added to the dried extract and the mixture was shaken at 37°C for 2 h. Subsequently, 70 μL of N-methyl-N-(trimethylsilyl) trifluoroacetamide was added to the methoximated samples, which were then incubated at 30°C for 30 min. The derivatized samples were transferred to GC vials and sealed tightly with moisture-proof Parafilm. All chemicals and reagents used in this study were analytical grade and were not further purified.
GC-MS analysis and data processing
The GC–MS analysis and data-processing were performed according to our previously described procedures (Nam et al., 2015, 2016). Six biological replicates grown under either salt- or non-stressed conditions were randomly analyzed. Each sample (1 μL) was injected into the GC–MS (Clarus 680/600T; Perkin-Elmer, Waltham, MA, USA) through a DB-5 MS capillary column (30 m ´ 0.25 mm i.d., 0.5 μm particle size; J&W Scientific, Folsom, CA, USA). The injector was operated in the split mode at a ratio of 10 : 1 and an injection temperature of 250°C. Each sample was introduced at an initial oven temperature of 60°C and held for 2 min, then increased at 5°C min-1 to a final temperature of 320°C and held for 11 min. The total run time was 65 min. Helium was used as the carrier gas, with a flow rate of 1.2 mL min-1. The mass data were collected in the electron impact mode with 70 eV ionization energy, and were recorded from 44 to 620 m/z with a scan time of 0.15 scans s-1. The ion source and interface temperatures were 250°C and 280°C, respectively.
The resulting GC–MS data were processed with TurboMass software (version 5.4.2.1617; Perkin-Elmer). A total ion chromatogram was acquired from the EI-scan by time. Peaks with signal-to-noise ratios < 200 were discarded and conjugated peaks were de-convoluted into one single peak by the software. For metabolite identification, peaks were compared with a customized reference spectra database from the National Institute of Standards and Technology (USA), based on retention indices and mass spectral similarities. Most of the constituents were further verified by matching the mass spectra of obtained metabolites to authentic reference standards. All peaks were then normalized using ribitol as an internal standard and exported into an Excel data sheet for statistical analysis.
The resultant datasets were subjected to multivariate statistical analysis, using SIMCA-P+ software (version 12.0; Umetrics AB, Kinnelon, NJ, USA). All data were Pareto-scaled and an unsupervised principal component analysis (PCA) was conducted to explore dataset variations.
Compositional analysis of rice grains
For rice grains, all proximates were determined according to the methods specified by the Association of Official Analytical Chemists. Moisture content was recorded based on weight loss after the grain samples were oven-dried at 105°C (AOAC, 2000). Crude protein was analyzed by the Kjeldahl method (AOAC, 2005) and crude fat was obtained through Soxhlet extractions (AOAC, 2006). Crude ash was estimated after dry-ashing in an oven at 600°C (AOAC, 2005). Crude fiber was determined by successively digesting each sample with sulfuric acid and sodium hydroxide solutions (AOAC, 2006). Carbohydrate concentrations were calculated by subtracting the total percent values of crude protein, crude fat, and crude ash from 100. All essential amino acids, except tryptophan, were evaluated by an automatic amino acid analyzer (Biochrom 30 Amino Acid Analyzer; Biochrom, Cambridge, UK) after hydrolysis with hydrochloric acid according to the method specified by the Korean Food Code (MFDS, 2011). The level of tryptophan was determined after hydrolysis with a sodium hydroxide solution, using a high-performance–liquid chromatography (HPLC) system (1100 Series; Agilent, Santa Clara, CA, USA). Fatty acid compositions were measured after lipid extraction with hydrochloric acid and diethyl ether (AOAC, 2005), using a GC instrument (7890 GC; Agilent). Determinations of minerals were conducted with an inductively coupled plasma-optical emission spectrometer (Optima 8300; Perkin-Elmer) and an ultra (UV)-visible (VIS) spectrophotometer (Optizen 2120 UV Plus; Mecasis, Daejeon, Korea) after digestion with nitric acid, per the procedure stipulated by the Korean Food Code (MFDS, 2011). Vitamins B1 and B2 were extracted from the powdered samples with ammonium formate according to the method proposed by Martins-Junior et al. (2008). Their concentrations were obtained via liquid chromatography–tandem mass spectrometry (API 4000; AB Sciex, Framingham, MA, USA). For the analysis of vitamin E, samples were saponificated, extracted, and analyzed by HPLC (1100 Series; Agilent) (AOAC, 2005). Phytic acid concentrations were determined using a method modified from that of Wheeler and Ferrel (1971). Samples were extracted with trichloroacetic acid and analyzed by the UV/VIS spectrophotometer at 480 nm.
All data obtained were analyzed using STATISTICA (version 8.0; StatSoft Inc., Tulsa, OK, USA). The statistical significance of differences in the means for each experimental group was calculated with Student’s t-tests. Mean differences were considered significant at p< 0.05.
Results and Discussion
Phenotypic changes in rice plants in response to salt stress
To test the effects of salt stress on rice growth, we monitored phenotypic changes in plants exposed to 51 mM NaCl during various developmental stages. At the tillering stage, the visual phenotypes differed significantly between salt-stressed and unstressed plants (Table 1). The former were significantly shorter (by 6.0%) and their shoot FWs and DWs were 16.9% and 15.3% lower, respectively. Likewise, root FW values were 13.2% lower for those stressed plants. During heading and ripening, heights were 4.5% and 4.8% lower, respectively, for salt-stressed plants but no differences in FWs or DWs were found between treatment types. Therefore, our results indicated that salt stress affects the development of rice shoots and roots at the tillering stage, but the influence is negligible at the heading and ripening stages. We also found that no differences in grain length, width, and 100-grain weight between salt-stressed and unstressed rice plants (data not shown).
Salt stress-induced metabolic alterations in rice plants during different growth stages
To explore the metabolic responses in salt-stressed rice, we performed untargeted GC–MS metabolite profiling according to growth stage and organ type. In all, 61 metabolites were identified in the shoots, roots, and grains during different stages (Table 2). These metabolites consisted of amino acids, fatty acids, organic acids, sugars, sugar alcohols, sterols, etc. At the tillering stage, the PCA score plot separated the two clusters of salt stress and non-salt stress conditions in shoot metabolites (Fig. 1A) and also revealed a clear discrimination between treatments in the roots (Fig. 1C). The PCA loading plot revealed that fructose and glucose greatly influenced the separation by PC 1 (55.9% of the total variance), while sucrose and phosphate were the main contributor to the separation by PC 2 (21.6% of the total variance) for the shoots (Fig. 1B). Minor contributions of γ–aminobutyric acid, pyroglutamic acid, shikimic acid, serine, and aspartic acid were also found. For the roots, the PCA loading plot indicated that fructose and glucose were the main contributors to the separation by PC 1 (40.6% of the total variance) and PC 2 (30.6% of the total variance) (Fig. 1D). Higher levels of glucose, γ–aminobutyric acid, aminoethanol, fructose, and arabinose were detected in salt-stressed roots, whereas phosphate, maleic acid, and sucrose were more abundant in unstressed roots. During heading, shoot metabolites did not differ noticeably between salt-stressed and unstressed conditions (Figs. 2A and 2B). For the roots, metabolites in stressed plants were markedly distinct from those of untreated plants (Fig. 2C). The major contributor to the division by PC 1 (46.9% of the total variance) was sucrose, whereas fructose was the primary contributor on PC 2 (29.3% of the total variance) for the roots (Fig. 2D). Glucose, γ–aminobutyric acid, aminoethanol, succinic acid, valine, trehalose, glycine, and benzoic acid were also contributed to the division by PC1 or PC2. At the ripening stage, no significant differences in metabolites due to salinity status were found in the shoots, roots, or grains (Fig. 3).
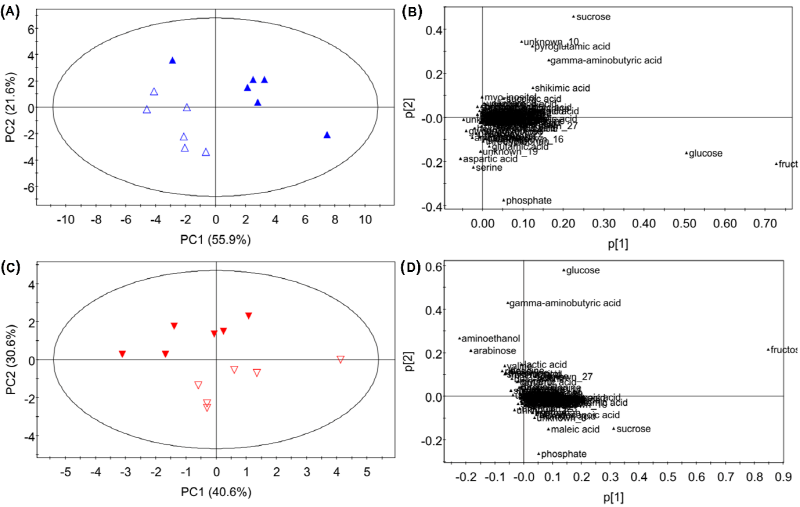
Fig. 1. PCA analysis of GC–MS data for rice shoots (▲, △) and roots (▼, ▽) at tillering stage when grown under salt stress (filled symbols) or non-salt stress (open symbols) conditions. Scores and loading plots from shoots (A, B) and roots (C, D). Ellipses represent Hotelling T2 with 95% confidence in score plots.
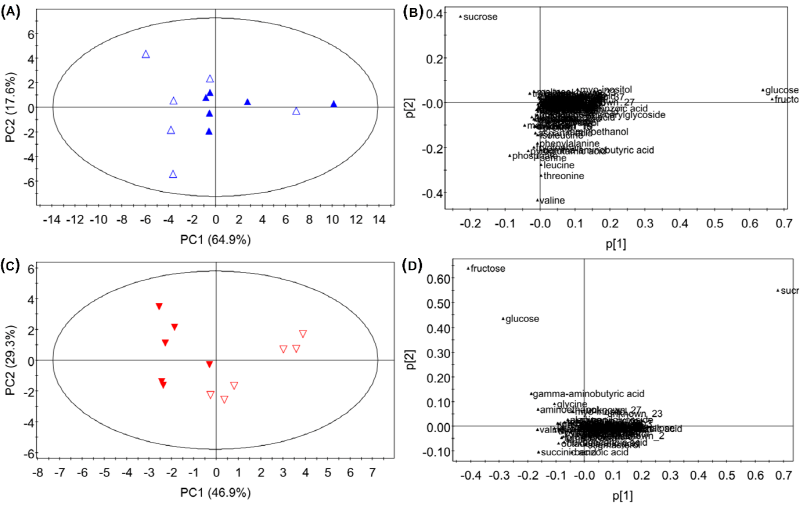
Fig. 2. PCA analysis of GC–MS data for rice shoots (▲, △) and roots (▼, ▽) at heading stage when grown under salt stress (filled symbols) or non-salt stress (open symbols) conditions. Scores and loading plots from shoots (A, B) and roots (C, D). Ellipses represent Hotelling T2 with 95% confidence in score plots.
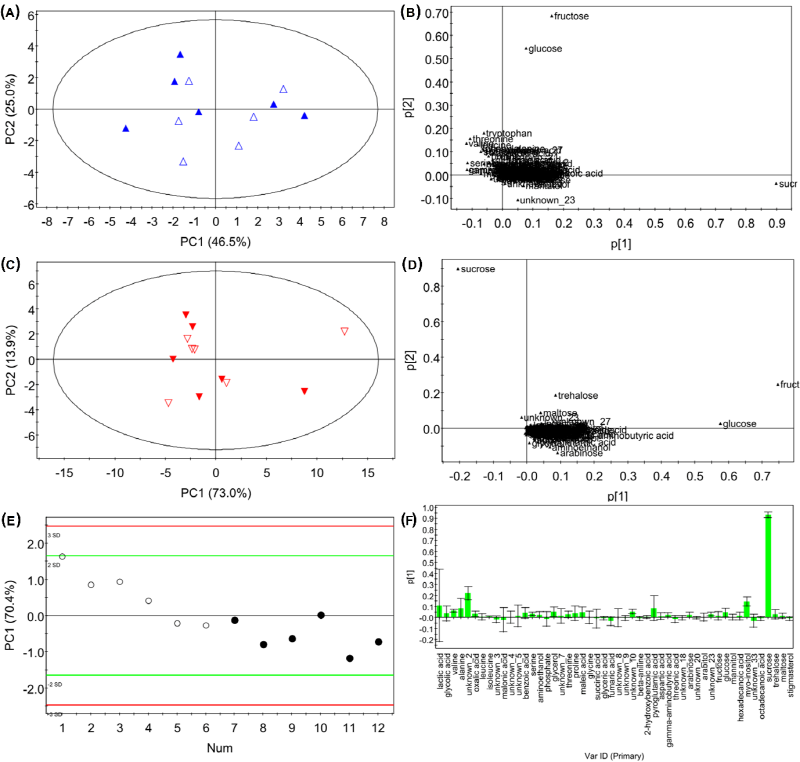
Fig. 3. PCA analysis of GC–MS data for rice shoots (▲, △), roots (▼, ▽), and grains (●, ○) at ripening stage when grown under salt stress (filled symbols) or non-salt stress (open symbols) conditions. Scores and loading plots from shoots (A, B), roots (C, D), and grains (E, F). Ellipses represent Hotelling T2 with 95% confidence in score plots.
Our PCA data suggested that variations in rice metabolites between salt-stressed and unstressed conditions are largely influenced by growth stage and organ type. Furthermore, these metabolic differences due to salinity can be attributed to sugar levels. In plants, sugars are closely associated with the regulation of metabolism, stress responses, growth, and development over the entire life cycle (Rolland et al., 2002). Soluble sugars in particular have a strong influence on the mechanism that enables tolerance to various abiotic stresses (Rosa et al., 2009). Under salinity conditions, soluble sugars contribute to osmotic balance, carbon storage, and the scavenging of free radicals (Parida and Das, 2005). The imposition of salt stress causes total soluble sugars to accumulate and starch to be depleted in stressed tissues (Parida et al., 2002). These changes in soluble sugar levels are variable among species and organ types and are dependent upon developmental processes. In the present study, we detected sugar-related metabolic alterations in salt-stressed shoots and roots at the tillering stage and in roots at the heading stage. However, metabolites in the shoots, roots, and grains could not be clearly discriminated by salinity during the ripening stage. These findings suggested that, over time, the influence of salinity on rice metabolites is reduced, especially for the grains.
Salt stress-induced compositional changes in rice grains
Analysis of the nutritional composition in plants exposed to stressful conditions is worth considering when evaluating quality and food value (Nam and Kim, 2015). For example, the application of long-term salt stress to plants of strawberry (Fragaria × ananassa) can improve fruit quality by enhancing the contents of antioxidants and soluble solids (Keutgen and Pawelzik, 2008). Exposure to low or moderate salinity stress stimulates the yield of essential oils from coriander leaves, which are widely used as food flavoring (Neffati and Marzouk, 2008). We examined the nutritional profiles that occur in grains from rice plants exposed to 51 mM NaCl in the irrigation solution. Although salt stress did not influence the concentration of individual proximates, amino acids, or vitamins in the grains, levels of linolenic acid (18 : 3) and tricosanoic acid (23 : 0) were 3.4% and 4.2% lower, respectively, as a result of such treatment, while concentrations were considerably higher for copper (by 26.8%), sodium (343.0%), and phytic acid (9.9%) in samples from stressed plants than from non-stressed plants (Tables 3 - 7).
Table 4. Amino acid compositions (g/100 g) for brown rice from plants grown under non-salt and salt treatments. ![]() |
|
Data are means (n = 3) ± standard deviations. p-values are from Student’s t-tests. |
Fatty acids are one of the most important constituents of cell membrane lipids, which play a fundamental role in permeability and regulation of fluidity (Los and Murata, 2004; Ashraf and Ali, 2008). Salt stress leads to modifications in their composition. For example, high salinity diminishes the ratio of unsaturated to saturated fatty acids in coriander leaves, which then decreases membrane permeability (Neffati and Marzouk, 2008). However, the extent to which membrane stability and permeability are reduced is lessened in salt-tolerant genotypes of Triticum aestivum and Brassica napus, respectively, under high salinity (Sairam et al., 2005; Ashraf and Ali 2008). Moreover, relatively lower membrane permeability is correlated with higher activities by antioxidant enzymes in such tolerant genotypes (Ashraf and Ali, 2008). We also noted that the levels of linolenic acid (18 : 3) and tricosanoic acid (23 : 0) were significantly decreased by NaCl stress in rice grains (Table 5). Accordingly, this alteration in the amounts of some fatty acids may have helped stabilize the membranes and alleviate the negative effects of salt stress.
When NaCl stress is induced, changes in mineral composition are pronounced in many plant species, as manifested by elevated concentrations of sodium and chloride but lower concentrations of calcium, potassium, and magnesium (Essa, 2002; Parida and Das, 2005; Keutgen and Pawelzik, 2008). Individual minerals are involved in regulating osmotic balance and turgor maintenance (Hu and Schmidhalter, 2005). Essa (2002) has reported that salt-tolerant soybean maintains lower leaf concentrations of sodium and chloride and a higher level of potassium when NaCl-salinity is increased. In contrast, the concentration of potassium in strawberry fruits is higher in salt-sensitive plants than in less-sensitive cultivars when excess NaCl is applied (Keutgen and Pawelzik, 2008). Our data also demonstrated that the amounts of copper and sodium in the grains were significantly elevated by salinity treatment while those of other minerals remained the same in those samples (Table 6).
Table 6. Mineral compositions for brown rice from plants grown under non-salt and salt treatments. ![]() |
|
Data are means (n = 3) ± standard deviations. p-values are from Student’s t-tests. |
Phytic acid is the major phosphorus storage compound in most seeds and cereal grains. It has long been regarded as an anti-nutrient for human diets because of its ability to chelate multivalent metal ions and, therefore, reduce the bioavailability of minerals in the digestive apparatus (Zhou and Erdman, 1995). Nevertheless, it also has a potentially positive role as an anti-oxidant and anti-cancer agent because it decreases ROS generation and lipid peroxidation. Doria et al. (2009) have reported that a low phytic acid mutant of Zea mays accumulates more free radicals but fewer γ-tocopherols when compared with the wild type. We also found that levels of phytic acid were higher in grains from stressed plants (Table 7), implying that the amount of this compound is increased so that plants might tolerate the oxidative stress caused by high salinity.
Conclusions
We examined the metabolic profile and nutritional composition of rice after salt stress was imposed. Treatment with NaCl in the irrigation solution significantly decreased shoot and root growth and induced metabolic alterations at the tillering stage. However, as development progressed from tillering to heading to ripening, metabolic variations associated with salinity diminished. Nutritional analysis of the grains revealed that the amounts of linolenic and tricosanoic acid were significantly reduced while those of copper, sodium, and phytic acid were enhanced in response to stress. Nevertheless, the differences were not great except for a four-fold increase in the sodium contents. These findings broaden our knowledge about metabolism in rice grains when plants are exposed to such challenges, and our data provide a basis for developing improved rice varieties with enhanced tolerance to abiotic stresses.