Introduction
Bovine respiratory disease (BRD) is a serious threat to the dairy and beef industry and has a considerable economic impact. It is most prevalently caused by bovine respiratory syncytial virus (BRSV) and bovine viral diarrhea virus (BVDV) (Griffin, 1997; Snowder et al., 2006; Brodersen, 2010; Oliveira et al., 2020). BRSV causes severe life-threatening infections, mostly in one- to six-month-old calves (Bryson et al., 1978; Kimman et al., 1988), and affects the respiratory epithelium of the nasopharynx, bronchi, bronchioles, and alveolar spaces, leading to pyrexia, anorexia, tachypnea, and dyspnea (Verhoeff et al., 1984). BVDV infection during pregnancy results in a variety of reproductive disorders (failure to conceive, stillbirth, abortion, and congenital deformity) and the birth of persistently infected (PI) calves (Grooms, 2004). PI calves are immunotolerant to BVDV and maintain the infection in herds. In practice, maternal antibody transfer is a widely accepted disease protection measure. However, as another commonly used disease management tool, vaccination before pregnancy could be a precautionary measure to control the disease. Commercial vaccines that consist of modified live or inactivated pathogens are in practice to prevent the negative impact of BRD; however, they have questionable efficiency (Theurer et al., 2015). Therefore, further study regarding the immunological aspect to develop a more efficacious vaccine is still needed.
A vaccine with adjuvants elicits the innate immune response via antigen presentation to T lymphocytes and promotes inflammatory reactions (Tizard, 2004) characterized by proinflammatory cytokines that ultimately impair cattle performance by increasing basal metabolism and tissue catabolism (Johnson, 1997). Lactating dairy cows experience an increased metabolic load, and vaccination at such a physiological loading stage further pushes them towards metabolic stress. Maternal immunization has the potential to protect dams, fetuses, and newborn calves during their first months of life until they are old enough to be vaccinated. In practice, lactating cows are immunized not only to prevent the spread of diseases on the farm but also to protect their offspring at an early age through transfer of the maternal immune response (Downey et al., 2013; Guzman and Taylor, 2015). In addition to serum, detecting antibody in milk is a convenient method for scrutinizing vaccinated cattle because antibodies against BRSV and BVDV in bulk milk or individual milk are used to monitor herd/individual infection status (Elvander et al., 1995; Lindberg and Alenius, 1999; Klem et al., 2014; Ohlson et al., 2014). Innate and adaptive immunity modulation after vaccination is reflected in serum and milk. Post-vaccination changes in milk components could provide information about their relationship to the immune response.
The purpose of this study was to evaluate the immune profiles in blood cells, serum, and milk after vaccination in dairy cows to improve understanding of the immunological profile generated by the inactivated vaccine in a field situation.
Materials and methods
Animals and vaccination
In total, twenty lactating dairy cows of different ages reared in the dairy farm were enrolled in the present study. Among these cattle, 8 were primiparous, 18 were multiparous with an average parity of 1.89, and no parity information was known for 2 cows. The mean age of the dairy cows in the experiment was 3.74 years. Cows were intramuscularly vaccinated with an inactivated vaccine (Barvac Elite 4-HS, Boehringer Ingelheim Vetmedica, Inc., St. Joseph, Missouri, USA) that contains infectious bovine rhinotracheitis (IBR) virus, BVDV type I, BRSV, Myxovirus parainfluenza type 3 (PI3), and Haemophilus somnus. Each dose of the vaccine consists of a virus over 2.5 log protection per 5 mL.
Sampling
Blood samples were collected via jugular venipuncture before vaccination on the same day and 14 days post-vaccination (dpv) in accordance with animal health regulations. The study was approved by the National Institute of Animal Science (NIAS), Rural Development Administration (RDA). For serum collection, blood was coagulated, followed by centrifugation at 1,800 × g for 20 minutes at room temperature; serum was then aspirated and aliquoted into 1.5 mL tubes. For RNA extraction, 3 mL of blood was drawn into a TempusTM RNA tube (Applied Biosystems, Austria) containing 6 mL of stabilizer. Both serum and blood tubes were stored below -60℃ until use. Individual milk samples were obtained both pre-and post-vaccination and stored below -50℃ until the assay was performed.
Immunoassay
Serum antibody responses against BRSV and BVDV were assayed by a competitive ELISA using an Ingenzim BRSV compac ELISA kit (Ingenasa, Madrid, Spain) and VDPro BVDV AB ELISA kit (Median Diagnostics Inc., Seoul, Korea) according to the manufacturer’s protocols. As a competitive ELISA, samples with higher antibody concentrations develop weaker color intensity. Antibody levels in milk samples were also evaluated by the same procedure.
The optical densty (OD) values of BRSV and BVDV antibodies were evaluated to compare with a positive control to determine the relative antibody concentration in the S/P ratio form according to the following equation.
S/P = (1)
Cytokines in both serum and milk were assayed using sandwich ELISA. Interleukin (IL)-1β concentrations were measured using a Bovine IL-1β Invitrogen Thermo Fisher Scientific kit (Vienna, Austria); IL-4 was evaluated using a Thermo Scientific kit (Waltham, MA, USA), and IL-17A was evaluated using a Kingfisher kit (Saint Paul, MN, USA). Likewise, the concentrations of interferon (IFN)-γ, tumor necrosis factor (TNF)-α, IL-2, and IL-6 were assessed by using a bovine kit from R&D Systems (Minneapolis, MN, USA). The concentrations were inferred using corresponding standards by measuring the OD value at 450 nm.
Milk constituents
An immunoperoxidase assay was performed to analyze milk samples for the presence of total immunoglobulin (Ig) A and total IgG following the manufacturer’s instructions (MyBioSource, San Diego, California, USA). Lactoferrin and lysozyme contents in milk were quantified using an ELISA kit developed by MyBioSource (San Diego, California, USA). Milk was centrifuged at 10,000 × g for 15 min at 4℃, the aqueous fraction was collected, and the cycle was repeated twice. Skimmed milk was used for the quantification of lactoferrin. Lysozyme was quantified from the milk centrifuged at 2,000 rpm for 10 min at 4℃. The final absorbance was measured at 450 nm using the ELISA plate reader, and the concentration was calculated based on the standards.
RNA extraction and quantitative real-time PCR
RNA was extracted from blood collected in the Tempus tube using TempusTM Spin RNA isolation reagent kit (Applied Biosystems, Thermo Fisher Scientific, Texas, USA) based on the guanidine hydrochloride method. Extracted RNA was quantified using a BioSpec-Nano Spectrophotometer (Shimadzu Biotech, Shanghi, China) at a 0.7-mm pathlength, and its purity was verified (OD260/OD280 absorption ratio > 2.0). Complementary DNA synthesis was performed using ReverTraAce qPCR RT kit (TOYOBO, Osaka, Japan) in a 10-μL reaction mixture using a template concentration of 0.5 μg·μL-1 by incubation at 37℃ for 15 min followed by 98℃ for 5 min and holding at 4℃ using Thermal Block All in one cyclerTM (Bioneer, Daejeon, Korea). The generated cDNA was diluted in nuclease-free water and stored at -80℃ until it was used for RT-qPCR. RT-qPCR was performed with the SYBR green premix (Bioneer, Daejeon, Korea) using the CFX ConnectTM Real-time system (BioRad, California, USA). A total of 17 primer pairs were used for amplification of the target genes, which are listed in Table 1. Genes included are Toll-like receptors (TLR) 1-10, myeloid differentiation primary response gene 88 (MyD88), retinoic acid-inducible gene (RIG-I), toll-like receptor adaptor molecule 2 (TICAM2), nuclear factor kappa B (NF-kB), melanoma differentiation-associated protein 5 (MDA5) and nucleotide-binding oligomerization domain 2 (NOD2). All primers were selected based on a published paper except for NOD2, which was designed on the IDT Realtime Design Tool platform. The housekeeping gene β-actin was used as the endogenous reference gene for the normalization of the Ct values of the target gene. The gene expression in fold change was evaluated using the 2-∆∆Ct method (Livak and Schmittgen, 2001).
Statistical analysis
The changes in the S/P ratios of BRSV and BVDV antibodies and cytokine concentrations pre- and post-vaccination were compared using a two-tailed, paired t-test. The correlation between cytokines including IL-1β, IL-2, IL-4, IL-6, IL-17A, TNF-α, and IFN-γ and BRSV and BVDV antibodies were evaluated using two-tailed Pearson’s correlation determined with GraphPad Prism version 7.04. The correlogram was computed and plotted using R software (R i386 3.6.2, R Foundation for Statistical Computing, Vienna, Austria). Correlations and differences were considered significant at a 5% level of significance (p < 0.05), highly significant at a 1% level of significance (p < 0.01), strongly significant at a 0.1% level of significance (p < 0.001), and very strongly significant at a 0.01% level of significance (p < 0.0001).
Results and Discussion
Antibody responses
Vaccination is the most important option for the control of BRD. Vaccination of lactating dairy cows with an inactivated vaccine resulted in a significant increase in specific antibodies against BRSV. The antibody concentration was interpreted from the S/P ratios analyzed using OD values. Screening of cows based on the cutoff values according to the manufacturer’s instructions showed that all were positive for both BRSV and BVDV pre- and post-vaccination, except one cow that was negative for BVDV before vaccination. The S/P ratio for BRSV increased within 14 dpv both in serum and milk, indicating an induced antibody response against BRSV as a result of immunization (Fig. 1). Monitoring for an increase in BRSV-specific antibodies in milk may be the best option to evaluate vaccine efficiency since milk is easily and regularly accessible. Thus, the parallel relationship in BRSV titers between serum and milk suggests that milk alone can be used for surveillance for BRSV antibodies (Ohlson et al., 2014). The ease of milk sampling from an individual or in bulk might have the potential for differentiating infected from vaccinated animals (DIVA) if specific antigenic vaccines are used.
Table 1. List of primers used in quantitative real-time PCR.
![]() |
F, forward sequence; R, reverse sequence. |
Before vaccination, BVDV-specific antibody levels were high in serum and vaccination didn’t further increase antibody levels in serum and milk (Fig. 1). The preexisting BVDV-specific antibodies in serum are the possible reason behind the non-significant increment of antibody levels. However, the higher BVDV-specific antibody levels in milk from infected cattle were successfully used to scrutinize and eradicate the disease in Sweden (Lindberg and Alenius, 1999). Thus, a lower concentration of BVDV-specific antibodies in milk in vaccinated than in infected cattle could be used as a diagnostic screening tool for DIVA.
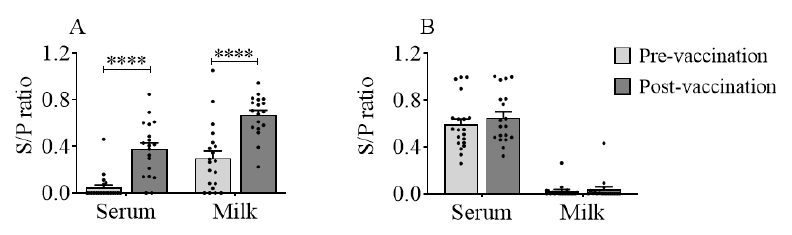
Fig. 1.Specific antibodies (sample to positive, S/P ratio) in lactating cows. Cows reared in a natural environment on the farm were vaccinated for bovine respiratory diseases (BRDs). Serum and milk samples were collected before and at 14 dpv, and specific antibodies were measured for (A) bovine respiratory syncytial virus (BRSV) and (B) bovine viral diarrhea virus (BVDV). Serum was diluted 50-fold for the BRSV S/P assay. For the remaining assays, samples were used without dilution. All data are expressed as the mean ± standard error of the mean (SEM). A two-tailed paired t-test was performed and asterisks (**** p < 0.0001) signify statistically significant differences.
Cytokine responses
Cytokine concentrations in serum and milk were measured to study if immune responses upon vaccination could modulate cytokine levels. IL-2, IL-4, and IFN-γ were mostly undetectable in both serum and milk regardless of vaccination. Interestingly, two major proinflammatory cytokines, IL-1β and IL-6 were significantly decreased upon vaccination in serum. However, both cytokines were not changed after vaccination in milk (Fig. 2). Another pro-inflammatory cytokine, TNF-α, was not detected in all except in one pre-vaccination serum sample. The concentration of the cytokine IL-17A was also negligible and not noted in immunized cows. Antiviral cytokine IFN-γ was expected to increase significantly but was upregulated in few cattle only.
BRSV either in live or inactivated or nano vaccine form significantly induced pro-inflammatory cytokines including IL-1β, IL-6, and TNF-α in bovine monocyte‐derived dendritic cells, macrophages, and epithelial cells (Werling et al., 2002; Guzman and Taylor, 2015; McGill et al., 2018). BVDV infected macrophages also induced IL-1β (Morales-Aguilar et al., 2020). In the present study, vaccination decreased both IL-1β and IL-6 levels in serum. The sampling after vaccination was done once at 14 dpv, while in most studies the samplings were performed within few days of infection, and were carried out in vitro. Thus, the natural farm condition and sampling time selection might result in such a deviation.
Downregulation of serum IL-1β was followed by a decrease in IL-2 since IL-1β and IL-6 regulate Th0 cells to differentiate into effector Th1 cells to produce IFN-γ and IL-2. In contrast, this decrease was masked in milk. The complete absence of IL-4 post-vaccination skewed the proliferation of Th2 cells. IL-4 stimulates B-cells for antibody production; however, a biased response of Th2 cells may predispose to increased pathology, whereas Th1 cells facilitate protection (Meyer et al., 2008). The inactivated vaccine developed humoral responses while inflammatory cytokine responses were downregulated.
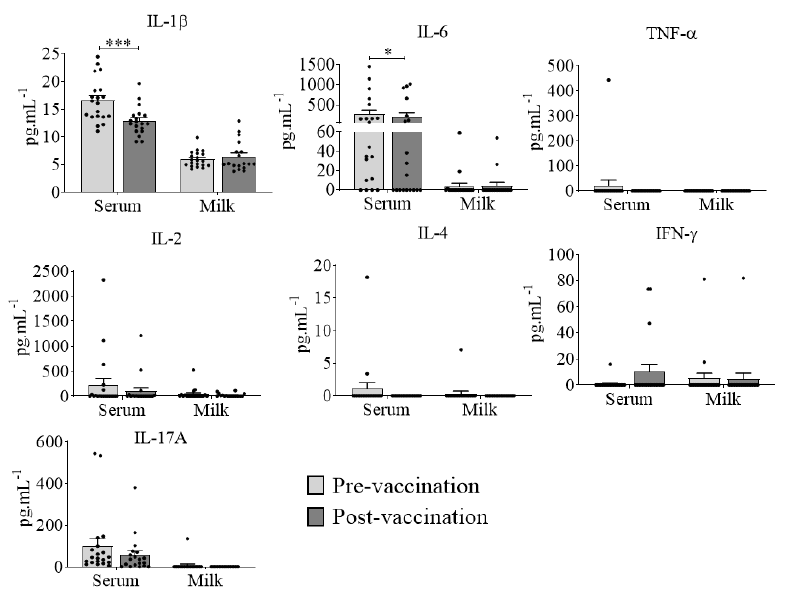
Fig. 2.Cytokine concentrations in response to vaccination. Serum and milk were sampled from cows reared at the farm pre- and post-vaccination. TNF-α was not detected except in pre-vaccinated serum samples, and IL-4 was not detected in post-vaccinated samples. All data are expressed as the mean ± standard error of the mean (SEM). A two-tailed paired t-test was assessed and asterisks (** p < 0.01) indicate a statistically significant difference. IL, interleukin; TNF,tumor necrosis factor; IFN, interferon.
Correlation between serum and milk
The constituents of serum and milk were compared to analyze their relationship. BRSV and BVDV antibodies in serum and milk were tallied to find corresponding relations. The correlation between serum and milk was strongly positive for BRSV-specific antibodies and moderately positive for BVDV-specific antibodies. Likewise, the correlation for IL-2 levels in serum and milk was very strong and in the same direction. A similar scenario was noted for IL-17A (Table 2). Since TNF-α was not detected in milk at all tested time points, performing a correlation study was not applicable. Although nonsignificant, the correlation between IL-1β levels in serum and milk was negative.
Studies regarding the bovine cytokine levels in milk and serum were done separately but the comparative analysis for vaccinated cows was not performed yet (Hagiwara et al., 2000; McGill and Sacco, 2020). Natural antibodies in bovine milk and blood plasma were highly correlated in a consistent and repeatable manner (Ploegaert et al., 2011). Nakajima et al. (1997) observed a strong correlation for IL-6 in between serum and milk in cows with naturally occurring coliform mastitis (Nakajima et al., 1997). The strong correlation between serum and milk for antibodies and cytokines in this study further strengthens the importance of milk as an alternative substitute for serological study.
Association of antibodies and cytokines
The correlation analysis among antibodies and cytokines was performed to assess the immune response following BRD vaccination in lactating cows. BRSV- and BVDV-specific antibodies in serum were negatively correlated with IL-1β. Although BRSV and BVDV infection-induced IL-1β production (Werling et al., 2002; Guzman and Taylor, 2015; McGill et al., 2018; Morales-Aguilar et al., 2020), vaccination downregulated it resulting in an inverse relation. The association between IL-1β and BVDV-specific antibodies in milk was reversed to that in serum; they were moderately positively related. The correlation between BVDV-specific antibodies and IL-2 was in the same direction; the association was stronger in milk than in serum. The association between BRSV-specific antibodies and IL-17A was positive in milk, and no significant relationship was found in serum. Generally, mastitis enhances pro-inflammatory cytokines in milk (Bochniarz et al., 2017) and vaccination has not been reported for changes in milk cytokine profiles. Thus, the relation between antibodies and cytokines indicates the requirement of further confirmation with more sampling time and multiple vaccination schedules.
Milk factors
Post-vaccination milk samples obtained 14 dpv revealed decreased levels of total immunoglobulin A (IgA), immunoglobulin G (IgG), and lactoferrin. The decrease was not significant but was on a trend of a significant difference for total IgA levels (Fig. 3). Lysozyme was not detected in any pre-or post-vaccination milk samples. The decreased total IgA, IgG, and lactoferrin levels were not associated with changes in BRSV- and BVDV-specific antibodies; however, IgG levels were weakly correlated with BRSV-specific antibodies (p = 0.059) (Table 3).
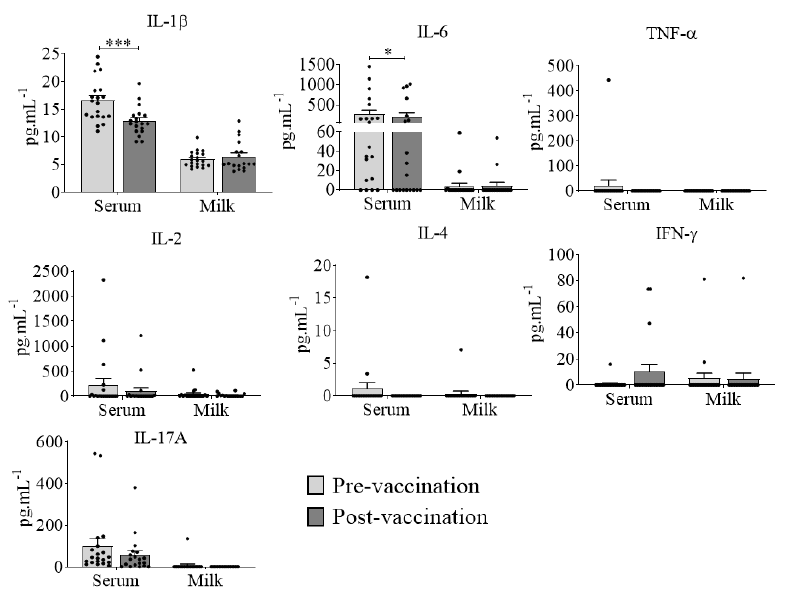
Fig. 3.Concentration of immune factors in milk. Total IgA, total IgG, and lactoferrin in milk were measured by ELISA pre- and post-vaccination (14 dpv) in dairy cows. Data are expressed as the mean ± standard error of the mean (SEM). A two-tailed t-test was conducted, and differences were significant at * p < 0.05. Ig, immunoglobulin.
Lactoferrin, an iron-binding glycoprotein, modulates the immune response through defense mechanisms by chelating iron required for microbial growth (Hao et al., 2019). It has strong antiviral potential against respiratory syncytial virus, rotavirus, herpesviruses, Mayaro virus, and other naked/enveloped DNA/RNA viruses (van der Strate et al., 2001; Carvalho et al., 2014). Decreased production of lactoferrin post-vaccination could be due to time variation as there was no correlation established with BRSV- and BVDV-specific antibody levels. Besides, various factors including the somatic cell count, stage of lactation, daily milk production, and parity affect the concentration of lactoferrin in bovine milk (Cheng et al., 2008). Vaccination had no influence on the total IgA in milk although it is an anti-inflammatory antibody required for mucosal defense against bacterial invasion (Cakebread et al., 2015).
Expression of pathogen recognition receptor (PRR) genes
The expression levels of different PRRs were studied in dairy cows immunized with an inactivated vaccine. Cytosolic receptor, NOD2 was significantly downregulated after vaccination, while RIG-I and MDA5 were slightly downregulated (Fig. 4). The decrease in expression of NOD2 in immunized cows may be critical for preventing uncontrolled inflammation in future encounters and may play a protective role against infection. Likewise, surface receptors including TLR1 and TLR5 were also downregulated. BRSV and BVDV infection induced the expression of TLR2 and TLR4 in bronchial lymph nodes (Tizioto et al., 2015) while the inactivated vaccination showed no such increment in blood cells.
Association of PRRs
BRSV-specific antibody was negatively correlated with NOD2 expression. NOD2 recognizes the viral ssRNA genome in the cytoplasm and utilizes the adaptor protein mitochondrial antiviral signaling (MAVS) to activate interferon regulatory factor 3 (IRF3) (Sabbah et al., 2009). It is essential for induction of both Th1- and Th2-type responses following costimulation with TLR agonists. The correlation of NOD2 with TLR4, TLR2, TLR5, and TLR7 supports its importance in a downstream signaling pathway.
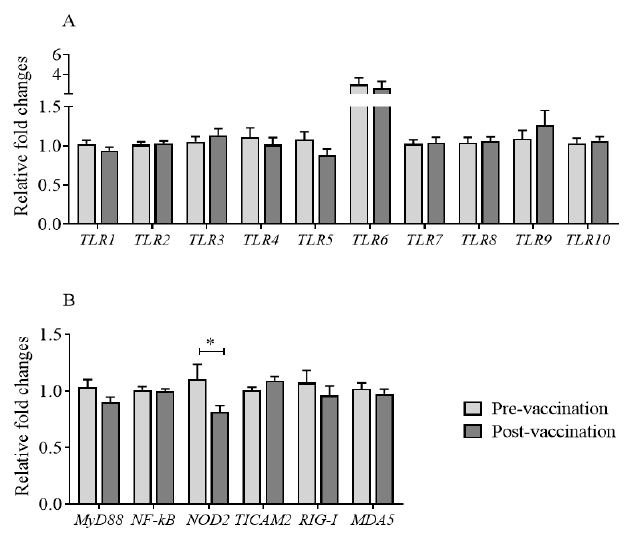
Fig. 4.Relative normalized expression of mRNA for (A) toll-like receptors (TLRs) and (B) adaptor proteins. RNA was extracted from the whole blood of bovine respiratory disease (BRD)-immunized lactating cows at pre- and post-vaccination time points. mRNA expression was measured using published and designed primers. Data are expressed as the fold difference in mRNA expression normalized to the housekeeping gene (β-actin) relative to the values obtained for pre-vaccinated cows using a two-tailed paired t-test where * p < 0.05 refer to a statistically significant difference. MyD88, myeloid differentiation primary response gene 88; NF-kB, nuclear factor kappa B; NOD2, nucleotidebinding oligomerization domain 2; TICAM2, toll-like receptor adaptor molecule 2; RIG-I, retinoic acidinducible gene; MDA5, melanoma differentiation-associated protein 5.
BVDV-specific antibody was moderately correlated with TLR2, TLR3, TLR4, and TLR5. Innate viral recognition of BVDV in cattle depends on TLR3 and TLR7 (Lee et al., 2008) and such viral recognition produces cytokines to stimulate inflammatory and adaptive immune responses (Janeway and Medzhitov, 2002; Schlender et al., 2005; Kawai and Akira, 2006). The correlation of BVDV-specific antibodies with TLR2, TLR3, TLR4, and TLR5 indicates their possible role in vaccination-induced immune responses. Although viral infection promotes TLRs to induce inflammatory cytokines such as TNF-α, IL-1β, and IL-6 (Kawai and Akira, 2006), BVDV viral infection down-regulated these cytokines to inhibit inflammation and enhances infection (Lee et al., 2008). The inactivated vaccine in this study slightly increased the expression of TLR2, TLR3, TLR7, and TLR8 and decreased IL-1β to control the inflammation.
Among PRRs, there were clusters of strong positive correlations among NOD2, MyD88, and TLR8 and among TLR1, TLR9, and TLR10. Likewise, TLR4, TLR2, and TLR7 were positively correlated with MyD88, TLR8, and NOD2 (Fig. 5). The formation of these clusters suggests their relationship to the immune response for cytokine production. Further, the slight increase in TICAM2 post-vaccination and its positive correlation with NF-kB favored the cytokines release pathway.
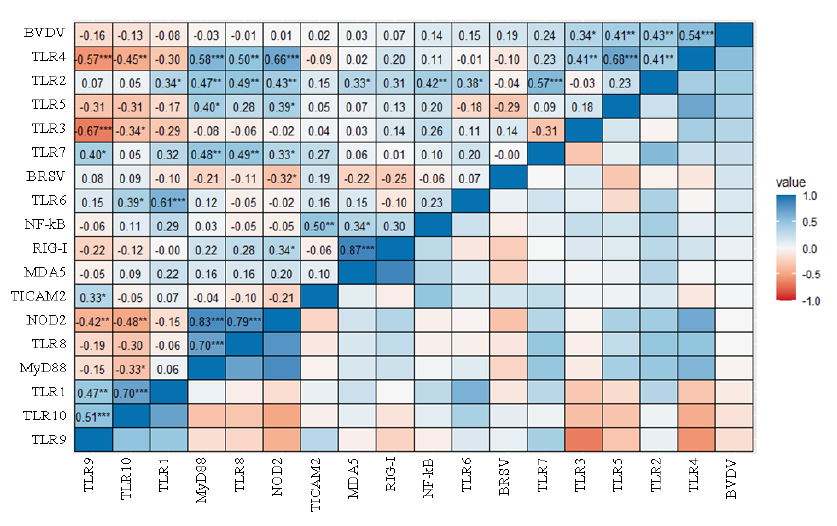
Fig. 5.Correlogram presenting the relationships among antibodies, pattern recognition receptors (PPRs), and cytokines in response to bovine respiratory disease (BRD) vaccination in cows. The numerical value and color denote the Pearson correlation coefficient and strength of the relationship, respectively, and asterisks (*p < 0.05, **p < 0.01 and ***p < 0.001) indicate statistically significant differences. BVDV, bovine viral diarrhea virus; TLR, toll-like receptor; BRSV, bovine respiratory syncytial virus; NF-kB, nuclear factor kappa B; RIG-I, retinoic acid-inducible gene; MDA5, melanoma differentiation-associated protein 5; TICAM2, toll-like receptor adaptor molecule 2; NOD2, nucleotidebinding oligomerization domain 2; MyD88, myeloid differentiation primary response gene 88.
TLRs and the other adaptor protein genes were not significantly modulated post-vaccination; thus, further evaluation within a few days after vaccination should be performed. However, the clustering of NOD2, MyD88, and TLR8 and the relationships of TLR2, TLR4, TLR7, and TLR8 with MyD88 suggest that MyD88-dependent activation of the signaling pathway is necessary for consecutive immune responses.
Conclusion
In conclusion, when dairy cows were inoculated with inactivated BRD vaccine, pathogen-specific antibody response, especially BRSV, was induced both in serum and milk. This result indicates that milk could be a better choice with an easier approach than serology for BRSV-specific antibody detection. Antibody response to BVDV was not induced by vaccination which was possible due to preexisting high-level antibodies and single-dose vaccination. PRR transcript analysis using RNA extracted from blood revealed that NOD2 expression was downregulated after vaccination. In addition, serum cytokine levels were not changed by single-dose vaccination except IL-1β. In the present study, sampling was done at a one-time point (14 dpv) and vaccination was also done once. Therefore, further studies with multiple sampling times and multiple vaccinations are necessary to characterize immune responses against BRD vaccination.