Introduction
Rice (Oryza sativa L.) is a prominent crop in agriculture and one of the leading staple foods for more than half of the global population (Tang et al., 2009) accounting for around 35 to 75% of the calorie intake (Krishnan et al., 2011). Rice is the second-most cultivated cereal crop worldwide and the average milled rice production is 496 million metric tons per year, which is grown on approximately 162 million hectares of land according to Statista data (Statista, 2021). However, increased production is needed to meet the demands of the rising global population, which is projected to be 59 to 98% from 2005 to 2050 (Valin et al., 2014). Furthermore, the rising population has stimulated more carbon emissions (Ren et al., 2015), which could be reduced with the continued growth of water-intensive rice crops.
Rice-growing areas with suitable climatic conditions are a prerequisite for increasing rice production (Kihoro et al., 2013; Khaitov et al., 2019). Desert soils contain low levels of organic matter and are considered economically unimportant (Skujinš, 1984), but they do potentially offer more space for food production to meet the global food demand. United Arab Emirates (UAE) is located in the Persian Gulf, and is one of the largest rice importing countries of the world. Growing rice in desert soil would be an alternative means of meeting the rising food demand in the country. Thus, UAE is focusing on extending rice cultivation in desert areas with the development of a special variety of rice (NN, 2020).
The water holding capacity of desert soils is lower than is required for growing rice. Haefele et al. (2009) suggest that approximately 500 - 1,000 liters of water are needed to produce one kilogram of rough rice. Alternately, Bouman (2009) mentions that about 2,500 liters of water is needed from either rainfall or irrigation in a rice field to produce one kilogram of rough rice, considering the evapotranspiration, seepage, and percolation. However, the availability of fresh and clean water remains a global challenge (Simonovic, 2002). In order to ensure sustainable rice production in desert soils, proper irrigation management with available water sources is needed.
Soils within UAE are sandy with low organic carbon and clay contents and are of low fertility (Hirich et al., 2020). Despite water shortages, unfavorable climatic conditions, and limited fertile soils, agriculture has become the second placed productive industry in UAE after oil (Power, 2014; Joseph, 2018). The agricultural land area in UAE is 397,000 hectares, which covers 4.75% of total land area. Until now, food availability remains a challenge owing to the low water-holding capacity of the soil (Aronovici, 1952). Ray (2020) states that UAE needs to grow rice in desert soils which could help to meet the growing food demand (Samad and Azar, 2019). It will also reduce the dependency on imported foods, and thus boost the country’s GDP. UAE also has a shortage of natural water sources (Hirich and Choukr-Allah, 2017), which hinders water resource management. Rizk and Alsharhan (2003) mentions the degradation of water quality, salinity, and depletion of aquifers, which also contribute to water shortage. Furthermore, the low rainfall and high evaporation rates (Murad et al., 2007; Mohamed et al., 2021) limit rain-fed agriculture in UAE; thus there is a need for artificial irrigation scheduling and management technology (Shahin and Salem, 2014). The Environment Agency (2017) states that the high withdrawal rates of groundwater with low recharge rates have contributed to a decrease in available water for irrigation. In addition, traditional irrigation methods, such as flooding have also led to the depletion of aquifers (Gonzalez et al., 2013). Moreover, for normal rice cultivation, a minimum rainfall of 1,150 mm and an average temperature of 20 - 27℃ are required. In UAE, the average temperature is 35 - 45℃ (May to October) and 15 - 35℃ (November to April), and the annual precipitation is around 60 - 100 mm. With this scarcity of water, irrigation is needed to grow water intensive crops in UAE, and improved automatic irrigation systems could save 60% of irrigated water usage (Frenken, 2009). Information and communication technology (ICT)-based irrigation along with water content sensors could supply specific amounts of water needed for rice cultivation in desert soils (Navarro-Hellín et al., 2016).
Manual irrigation scheduling for rice cultivation is difficult in desert soils owing to the poor water-holding capacity, labor shortage, and time taken; however, ICT-based water scheduling and management techniques would ensure the proper utilization of available water resources. Several researchers have introduced automatic irrigation scheduling techniques for agricultural purposes. Development and implementation of ICT-based irrigation system have been proposed, including an Internet of Things (IoT) platform using sensors and low-cost microprocessors, such as Arduino, and Raspberry Pi modules (Crespo and Iersel, 2011; Lakshmisudha et al., 2016; Rajalakshmi and Mahalakshmi, 2016; Prathibha et al., 2017; Akhter et al., 2018; Rao and Sridhar, 2018; Kim et al., 2019; Mehtre and Benni, 2020). Most of the systems have been used on small-scale irrigation sites for cultivating different plants and crops.
Therefore, there is a need to develop an ICT-based irrigation management system for the production of rice in desert areas. A sensor-based irrigation monitoring technology can precisely control irrigation scheduling in an experimental rice plot by monitoring soil water-holding characteristics. As a result, this study aimed to fabricate and evaluate the performance of a prototype ICT-based automatic irrigation monitoring system to explore rice cultivation in UAE desert soils. This newly developed technique could encourage farmers to cultivate rice and would assist in the remote monitoring of UAE desert environments.
Materials and Methods
Site description
This study was carried out from October 2019 to February 2021 at Al-Foah experimental farm site (Longitude 24°21'38"N; Latitude 55°48'05"E) at the UAE University (UAEU), Al-Ain, UAE. The experimental net house was constructed for rice cultivation in a desert environment. The total dimension of the net house was 315 m2 (length: 35 m × width: 9 m). It was split into four plots where a basic experiment was conducted on two plots for the sensor-based automatic drip and micro-sprinkler irrigation (Fig. 1). The sandy soils used in the experimental plots were considered to be representative of soil for rice cultivation in UAE desert areas. According to online weather observations, the experimental site presented moderate cold and very hot atmospheric conditions during the experimental period. The average temperature and humidity were recorded as 29 - 44℃, and 21 - 54%, respectively, in the Al-Foah experimental field area (WWO, 2020).
ICT-based irrigation monitoring system
The experiment was designed to monitor the automatic irrigation system using drip and micro-sprinkler methods. The study investigated the water holding behavior of sandy soil, light intensity, ambient temperature, and humidity inside the experimental net house. A data acquisition system (DAQ-system) was developed to monitor the experimental field data remotely. A schematic diagram of the ICT-based irrigation system is shown in Fig. 2. The irrigation system was supplied with a water reservoir, water pump, solenoid valves, relays, sand filter, and water flow meter. The pumping unit was assembled sequentially and the water pump supplied water to irrigate the rice plots through the water-flow pipes. A sand filter was used to clean sand particles from the irrigated water. A water flow meter measured the water flow rates during irrigation. A bypass line helped to remove excess water from the pumping unit. A manual control valve provided an option to control the water flow if it was required for manual irrigation.
An ICT-based automatic irrigation system was used to control the irrigation protocol in the rice plot according to the sandy soil water content percentages. Soil water content sensors were placed at five different depths of 10, 20, 30, 40, and 50 cm, respectively. In order to meet the water requirement, an automatic irrigation system was used, which watered the rice field accordingly. Two DAQ-boxes were installed inside the net house for monitoring the drip, and micro-sprinkler irrigation systems, separately (Fig. 1a and 1b). The DAQ-system sent the sensor data and actuator status to the developed web server via a data communication network for remote monitoring and storage.
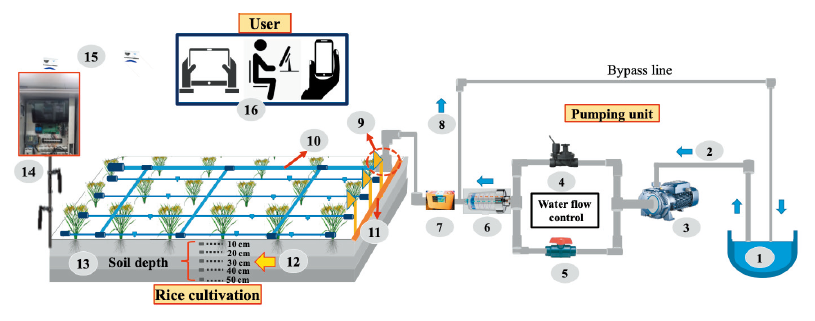
Fig. 2. Layout model of ICT (information and communication technology) based irrigation system for rice cultivation in UAE desert soil. Water source (1), water flow direction (2), water pump (3), solenoid valve (4), manual control valve (5), sand filter (6), water flow controller (7), bypass line (8), pipe joint (9), drip irrigation system (10), micro-sprinkler irrigation system (11), soil water content sensors (12), sandy soil plot (13), DAQ (data acquisition) box (14), data transferring (15), and data monitoring (16).
Fabrication of the data acquisition system
Material selection
The selection of hardware and software components is necessary to build a DAQ-system for real-time investigation of sensor outputs. The device and materials were selected based on the environmental conditions of the experimental site. The major components are shown in Fig. 3 and Table 1.
Raspberry Pi is a single-board micro-computer (Fig. 3a), which makes it comparatively easy, cheap, and suitable for collecting sensor data and controlling the actuators. Recently, it has been used to implement IoT functions in various agricultural fields (Iqbal et al., 2019; Kim et al., 2020). Raspberry Pi is equipped with Wi-Fi and Bluetooth modules that can be easily configured by connecting to a 7-inch display monitor (Fig. 3b). Pi supports various programming languages and provides good communication with other devices. It allows the use of a Linux-based Windows operating system, which is an open-source system that permits thousands of open source programs and features. Therefore, Raspberry Pi was selected as the main control device for the automatic irrigation system and for monitoring real-time data obtained from the sensor nodes.
The Raspberry Pi is embedded with 40 pins: 26 are GPIO (general-purpose input/output) pins which are used to interpret digital signals, and the remaining are power (up to 5 VDC) and ground pins. Since GPIO pins are unable to interpret analog signals directly, an 8-channel and a 10-bit analog to digital converter (ADC) (Fig. 3c) were used as microcontrollers for processing the analog output signals to digital. A configuration of the serial peripheral interface (SPI), 1-wire, and I2C (inter-integrated circuit) were made to communicate with the ADC chips and Raspberry Pi. Water content, light intensity, temperature, and humidity sensors were used to measure the data during the experiments (Fig. 3d - f). A 5V relay was used to control the 24 V DC water pump and solenoid valves (Fig. 3g - i).
DAQ box fabrication and sensor interfacing
The DAQ-box was constructed using sensor connections and a circuit board, a Raspberry Pi along with a display screen for data monitoring and control, electronic components, and a power supply. The components of the DAQ-box are shown in Fig. 4. The sensors were manually interfaced with the circuit board for better connections to achieve the most reliable sensor response. In a Raspberry Pi, the data communication bus includes MOSI (master out slave in), MISO (master in slave out), SCLK (clock for SPI communication), CE (chip enable), SDA (serial data), and SCL (serial clock) ports that were used to maintain communication with the sensors and actuators. A schematic diagram showing sensor and actuator interfacing with the ADC and Raspberry Pi is shown in Fig. 5.
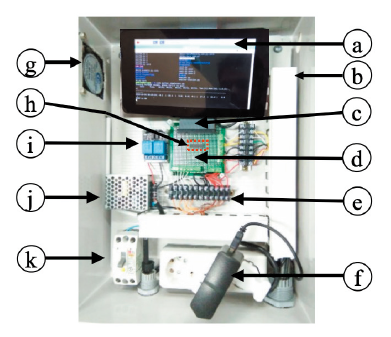
Fig. 4. Assembly of a DAQ (data acquisition) box for basic performance test. Raspberry Pi display screen (a), fabrication channel (b), ribbon cable (c), circuit board (d), sensor connectors (e), power supply (f), cooling fan (g), microcontroller (h), relays (i), voltage controller (j), and circuit breaker (k).
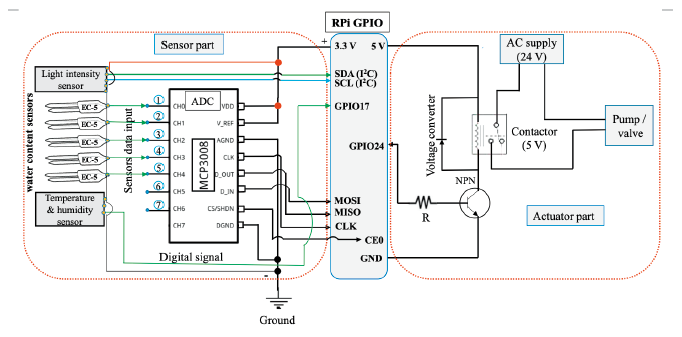
Fig. 5. Schematic diagram of sensor and actuator interfacing with the ADC (analog to digital converter) and Raspberry Pi. GPIO, general-purpose input/output; SDA, serial data; I2C, inter-integrated circuit; SCL, serial clock; MOSI, master out slave in; MISO, master in slave out; CLK, serial clock; CE0, chip enable; GND, ground; AC, alternative current; NPN, negative-positive-negative.
The ADC was also equipped with 16-channel ports where eight channels (CH0-CH7) were set up to read the analog input signal, and convert it to a digital signal through the SPI communication protocol. The other eight channels placed on the opposite side of the ADC, namely, Vdd (drain supply voltage), VREF (reference voltage), AGND (analog ground), CLK (serial clock), Dout (serial data out), Din (serial data in), CS/SHDN (chip select shutdown), and DGND (digital ground) were used to communicate with the Raspberry Pi GPIO pins. The sensor wires were connected with the 3.3 V GPIO pins of the Raspberry Pi, and the ADC provided 10-bit (1,023) output for the analog sensor, which might equal the input voltage of the sensor modules. The light intensity, ambient temperature, and humidity sensors delivered digital input signals. The soil water content sensors used in the experiment generated analog signals. However, the soil sensors were unable to be connected to the Raspberry Pi GPIO pins directly; therefore, the ADC converted the analog signal to digital to facilitate this connection. In addition, relay modules were used to operate the water pump and the solenoid valve to control the flow of irrigation water through the pipes.
A power converter was used to supply the required power to regulate the relay module connected with the Raspberry Pi GPIO pins. The ground and power ports of the sensors and actuators were connected to the Raspberry Pi power and ground pins along with the assigned GPIO pins to make circuit connections. A python language-based program was used to control the water pump and solenoid valve based on the soil water content. The Raspberry Pi also displayed sensor values and pump status on the screen, and stored them in an assigned folder.
Experimental and analytical procedure
Installation and performance evaluation of an ICT-based DAQ-system
A fabricated DAQ-box was installed in the rice-growing plots inside the net house. Five soil water content sensors were placed at five different depths (Fig. 6a) for monitoring the water content in the desert soil. The soil water content sensors were calibrated using a calibration equation provided by the manufacturing company. According to the sensor manual, the output of the sensor (mV) multiplied by 11.9 × 10-4 and subtracted by 0.401. The values displayed in volumetric water content percentages with a maximum at ≥ 60% in pure water, presented the real-time data on a scale of 0 to 100%. Furthermore, light intensity, temperature, and humidity sensors were placed in the middle of the experimental plot to investigate the environmental characteristics inside the net house. All the sensors were connected to the developed DAQ-system.
The main dripper line was arranged laterally with a 30 cm spacing for drip irrigation, and the emitter spacing was 10 cm (Fig. 6b). For micro-sprinkler irrigation, a 3 m interval spacing was set for the micro-sprinkler support pipe in the experimental plots. The VAN series arc (0 - 180°) spray nozzles were selected with 10° trajectories for spraying the irrigation water. The irrigation protocol was designed to ensure water coverage up to 2.1 bar water pressure.
An irrigation logic was developed and used to control the ICT-based irrigation system for the experimental rice plots, depending on the real-time sensing values through the commercial soil water content sensors (Fig. 6c). A Raspberry Pi regulated the sensor-based automatic irrigation system according to the preferred water application rates. In the experiment, the irrigation logic worked depending on the Raspberry Pi decision; the program was executed according to the output response of the five water content sensors placed at different depths, and calculated the average values following the input command. The desired water level was fixed at 30% for rice cultivation in the desert environment. If the water content was lower than the required value, the pump and valve automatically turned on to deliver the target irrigation. If the water level exceeded the fixed limit, the actuators automatically stopped the irrigation.
Data communication through a wireless network and storage
A wireless network system was used for real-time monitoring of the sensor values and for remote control of the irrigation system. Eight sensor nodes (five soil water content sensors, plus light intensity, ambient temperature, and humidity sensors) were linked to the Raspberry Pi GPIOS to obtain sensor data from each individual experimental plot (Fig. 7). The data were posted and stored in a Linux-based VPS web server. Soil water content data were used to control the irrigation pump based on irrigation requirements.
The performance of these systems depended on the capability of the Raspberry Pi. Data were collected, converted, filtered, and stored in a local database. A RESTful (representational state transfer) service was used to store data in the database in JSON (Javascript object notation) format. A web application service was created to receive the data and show real-time sensor readings and irrigation status in the developed DAQ-system. During this process, sensor-based data were collected and monitored throughout the experimental period; it was downloaded from the webserver and analyzed to evaluate the overall performance of the DAQ-system.
Results and Discussion
Sensor data monitoring and storage
Fig. 8 shows the overall installation setup and performance of the ICT-based irrigation monitoring system integrated with the Raspberry Pi for growing rice in UAE desert soil. Soil water content, light intensity, temperature, and humidity sensors were used to record the water content and the climatic behavior inside the experimental net house. Soil water content sensors measured the water content at 10 cm intervals down to a 50 cm depth, which defined the sandy soil water consumption characteristics. The irrigation pump and valves were controlled together by taking the average value of the five water content sensors. The systems followed the pre-set irrigation requirement for growing rice in desert soil, and successfully performed drip- and micro-sprinkler irrigation based on the developed irrigation logic. Furthermore, the climate sensors recorded the ambient environmental characteristics such as sunlight, temperature, and humidity inside the experimental net house.
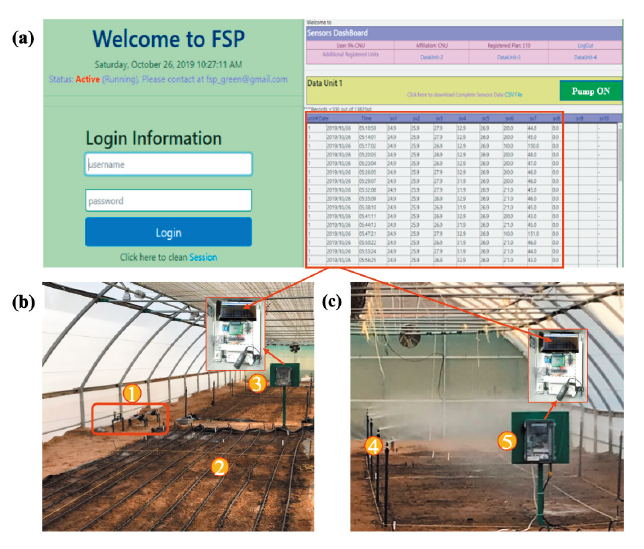
Fig. 8. Installation of DAQ (data acquisition) system for monitoring the irrigation of rice growing plots in desert soil inside the net house. Webserver dashboard snapshot (a), drip and micro-sprinkler irrigation monitoring plot (b, c): pumping unit (1), drip line placement (2), DAQ-system installation (3, 5), and microsprinkler irrigation (4). FSP, file service protocol.
After assembling the ICT-based irrigation management system, the reading of the sensors and actuator status was checked in the Raspberry Pi terminal. The results were displayed in the Raspberry Pi monitor for onsite monitoring; at the same time the data were sent to the web server for remote monitoring of the real-time situation of the experimental rice plots. Communication reliability depended on an available internet connection and successful program activities while the data were sent to the webserver. A commercial 4G pocket Wi-Fi module (DWR-932M, D-Link, Taiwan) was connected with the Raspberry Pi port that activated the Pi embedded Wi-Fi and helped to send the sensor output to the dedicated web server for monitoring irrigation performance (Fig. 8a - c). In the developed web server, the sensor dashboard stored the collected data in an assigned folder. The indicted columns present the eight sensor outputs in respect to the standard time zone and according to the ADC serial connection with the sensors. It was possible to monitor and download the data as a CSV file from anywhere around the world using the assigned URL (uniform resource locator). All sensors and actuator outputs were monitored and stored at 3 min intervals during the experimental period. Sometimes, the system failed to send the sensor data to the webserver owing to no internet connection, but the Raspberry Pi automatically backed up the data and saved it in an accredited folder using python programming logic. The python program was executed successfully and made to run the irrigation system and monitor the sensor results automatically with real-time water pump status on the Raspberry Pi terminal.
Soil water content variations at different depths in desert soil
Rice plants are unable to uptake water from deep soil layers owing to their shallow root system (Kondo et al., 2000). Thus, the evaluation was extended to compare the water content capacity of different layers within desert soil. The investigation results showed the diverse features of the soil water content at 10, 20, 30, 40, and 50 cm depths for rice cultivation in desert soil. The data were downloaded through the server access and analyzed for 5 months (October 2019 to February 2020) during the rice cultivation period. Graphical representations of the average results for drip and micro-sprinkler irrigation are shown in Fig. 9. Maximum water content values were found at 10 cm soil depth under drip irrigation owing to the shorter distance between irrigation source and medium-textured soil characteristics in the experimental plots. In addition, the water contents varied in different months owing to climatic variation. The average soil water content was recorded as 33.91 ± 2.15, 28.80 ± 1.03, 29.30 ± 2.75, 26.95 ± 1.35, and 27.27 ± 1.72% for drip irrigation, and 28.20 ± 1.08, 26.94 ± 1.48, 34.60 ± 1.77, 26.01 ± 1.87, and 31.26 ± 1.49% for micro-sprinkler irrigation at 10, 20, 30, 40, and 50 cm for the months of October 2019, November 2019, December 2019, January 2020, and February 2020, respectively.
Under micro-sprinkler irrigation, the results showed that soil water movement followed a trend of an increase in water percentage down to 30 cm, after which water percentages reduced at the maximum depths of 40 and 50 cm. This might have been because of the soil profile variations and textural characteristics in the experimental plots. In addition, the water was sprayed in a forward direction that covered the maximum area of the rice plot for the micro-sprinkler irrigation, while for drip irrigation, water dropped in a specific area for wetting the soil. This could have caused water content variations at the different soil depths. Moreover, in the individual depths, the water content percentages showed higher values in the months of December 2019 and January 2020 for drip irrigation. On the other hand, the soil water content varied in different depths of desert soil based on the irrigation methods. Fig. 9 (a, b) shows the variations in soil water content for drip and micro-sprinkler irrigation during the rice cultivation period inside the experimental net house.
At the drip irrigation site, significant variations of soil water content were found at the 10 cm depth during the experimental period, and the maximum and minimum average soil water contents were recorded as 36.38 ± 10% and 31.48 ± 1.23%, respectively (Fig. 10a). In this irrigation method, water was distributed with fewer variations at the 10 cm depth compared to the micro-sprinkler irrigation. On the other hand, there were significant variations among the 20, 30, 40, and 50 cm depths. Besides, in the micro-sprinkler irrigation, maximum water content was at 30 cm and 50 cm depths, and the same water distribution characteristics were shown in the experimental period due to the desert soil water holding disparity (Fig. 10b).
Rice yield depends upon a sufficient water supply to the root zone during the cultivation period (Kato et al., 2007); this improves rice field capacity and ensures proper growth and yield. Usually, rice is cultivated in heavy rainfall areas on clay to loamy soils with good water holding capacity. Sandy soils are unable to hold sufficient water due to the large particle sizes, therefore, it is a challenge to grow rice in a desert area. An automated DAQ-system was used to continuously monitor the soil water content (%) during the experimental period and to ensure a stable amount of irrigation in the rice plot. In the analyses, the mean values of the water content levels were significantly different (p < 0.05) in the five depths of soil among individual experimental months. The variations of the water content for drip and micro-sprinkler irrigation are shown in Fig. 10. Water content variations at the particular depths were very similar at 10 cm for drip, and 20 - 30 cm for micro-sprinkler irrigation; in the remaining depths, the water content variations were lower or higher owing to the daily conditions in different months and the differing soil wetting patterns of drip and micro-sprinkler irrigation.
Climatic characteristics inside the net house
Average data of light intensity, ambient temperature, and humidity inside the experimental net house were collected from the web-server storage and results are shown in Fig. 11. Sensor readings were recorded throughout the rice cultivation period in the desert soil environment. Solar radiation inside the net house increased up to noon and then began to reduce until sunset. The maximum average value was recorded as 7,160 lx, and the minimum value was 882 lx. The light intensity sensors showed relatively moderate values owing to the winter environment. The average light intensity results during the experimental period are shown in Fig. 11a.
Rice also needs sufficient sunlight for normal physiological activities. In addition, the temperature and humidity may affect rice production, especially in very hot and cold weather conditions. After sun rise at 06:30 h, the maximum light intensity values were recorded at 14:00 h, and the minimum intensity was observed at 17:45 h. The average daylight intensities were measured in lx and the values indicated that sunlight did not give direct heat to the experimental rice plants inside the net house, because the illuminance did not exceed 32,000 lx (PM, 2020). On the other hand, maximum temperature and humidity were observed at 45 ± 3.0℃, and 58 ± 2.0% in October 2019 and January 2020, respectively. In addition, the minimum temperature and humidity were recorded as 24 ± 3℃, and 50 ± 4% in January 2020 and November 2019, respectively. A graphical presentation of temperature and humidity is shown in Fig. 11b. The results showed the inverse relationship between temperature and humidity; when the temperature increased, humidity decreased throughout the experimental period inside the net house. The maximum and minimum temperature variations inside the net house were found to be slightly higher than the outside climatic recorded values (website data not shown). This may have occurred because of the closed atmosphere inside the experimental net house.
Conclusions
A prototype DAQ-system was developed, installed, and evaluated to control irrigation for rice cultivation in a UAE desert soil. Sensor-based technology was used in this study; it reduced human interaction, saved time and cost, and improved water usage efficiency in the experimental rice plot. The prototype was fabricated using a wireless communication network of an actuator and sensor, a database web-server, a small-sized low-cost single-board Raspberry Pi, and a simplified program with easy maintenance. An ICT-based operating system controlled the sensor and actuator activities and was responsible for timely irrigation by controlling the solenoid valves through relay (on/off) activities. Though the existing system performed well for the small-sized experimental rice-plot in the desert soil environment, further improvement is needed to make the system more reliable and adaptable for commercial applications. Likewise, a large-scale irrigation management system is required and highly recommended for growing water-intensive crops in desert areas. To accomplish this purpose, an IoT-based control system connected to mobile applications could be used. A combined program with a data communication module and collection device should be devised for satisfying the digital and analog output as well as assuring a proper signal and power supply. In the current experiment, a commercial IoT cloud platform was used, and it is suggested that it would be more efficient to design and implement its web database station to integrate rice cultivation environment data, production, and cultivation history into producer to consumer. This would be advantageous for distributing records along with the developed DAQ-system among entrepreneurs and farmers to create a base station for rice cultivation in desert soil.
Acknowledgments
This work was carried out with the support of the “Cooperative Research Program for Agriculture Science and Technology Development (Project No. PJ014538022020)” Rural Development Administration, Republic of Korea.
Authors Information
Mohammod Ali, https://orcid.org/0000-0002-1822-3005
Md Nasim Reza, https://orcid.org/0000-0002-7793-400X
Shafik Kiraga, https://orcid.org/0000-0002-7157-5288
Md Nafiul Islam, https://orcid.org/0000-0002-1980-2148
Milon Chowdhury, https://orcid.org/0000-0002-9887-7980
Jae-Hyeok Jeong, https://orcid.org/0000-0002-0888-0554
Sun-Ok Chung, https://orcid.org/0000-0001-7629-7224