Introduction
Chemical pesticides have been extensively utilized in agriculture worldwide over the past few decades. This has significantly increased environmental pollution and poses health risks due to their potent toxicity and propensity to accumulate in various ecosystems (Alengebawy et al., 2021). Among these chemicals, organophosphorus pesticides (OPs) stand out as one of the most commonly used synthetic pesticides globally, with over 200 varieties in use for various purposes (Kushwaha et al., 2016). Unfortunately, many of these OPs are associated with acute and sub-acute toxicity in animals, leading to adverse clinical effects, such as immunotoxicity, impaired reproduction, endocrine disruption, cellular damage, and increased oxidative stress (Walker, 2003; Gupta et al., 2017; Sule et al., 2022). Despite certain developing countries banning or imposing restrictions on the use of organophosphates due to concerns about their detrimental effects, these chemicals can persist in the environment, continuing to expose organisms and leaving them susceptible to pesticide-induced reproductive hazards (Pathak et al., 2022). One such pesticide is chlorpyrifos (CPF), which is moderately toxic and has a half-life of 10 - 120 days. One of its important metabolites is 3, 5, 6-trichloro-2-pyridinol (TCP), which has a half-life ranging from 65 to 360 days in the soil (Li et al., 2020). As a charged molecule with higher water solubility than CPF, TCP can easily leach into the groundwater and surface water, causing widespread contamination of the soil and aquatic systems (Li et al., 2010; Lu et al., 2013). Recent studies highlight the impact of continued exposure to OPs like chlorpyrifos on reproductive functions. Both chronic and acute exposure to these chemicals and their metabolites have been linked to disruptions in the endocrine balance, potentially leading to adverse effects on the reproductive function, embryonic development, offspring health, and metabolic well-being (Dutta and Sahu, 2013; Ventura et al., 2016; Dziewirska et al., 2019). Pesticides can directly damage the structure of cells in the male reproductive system. This damage can interfere with normal cellular functions, disrupt hormone signaling, and potentially lead to reduced sperm production, impaired sperm quality, and overall dysfunction of the reproductive organs (Rey et al., 2009; Zhou et al., 2019). Rats that were subjected to chlorpyrifos exhibited a significant reduction in the number of spermatogenic cells within the seminiferous tubules resulting in a reduction in sperm count (Elsharkawy et al., 2014). Moreover, previous studies found that direct exposure of boar spermatozoa to chlorpyrifos negatively affected their morphology and functional abilities (Adikari and Yi, 2022). Despite growing concerns, only a limited number of in vitro studies have investigated the effects of pesticide metabolites on the sperm function and fertility of livestock. Therefore, in this study, we examined the effect of direct exposure to TCP, a metabolite of chlorpyrifos, on boar sperm function.
Materials and Methods
Sperm preparation
Liquid boar semen samples were purchased from a local artificial insemination (AI) center. Samples exhibiting more than 80% sperm motility were used for the experiments. Boar spermatozoa were washed and then suspended in Beltsville thawing solution (BTS); (Pursel and Johnson, 1976) followed by incubation without TCP (or with 0.1% dimethyl sulfoxide [DMSO]; a vehicle control) or with TCP at concentrations varying between 10 - 200 µM, at 37℃ for 30 min and 2 hrs. Different concentrations of TCP (PESTIANAL, #33972, Sigma-Aldrich, Inc., USA) were prepared by dissolving it in DMSO.
Unless otherwise mentioned, all other reagents used in this study were purchased from Sigma-Aldrich Chemical Co., LLC (USA).
Assessment of sperm motility
A computer-assisted sperm analysis (CASA) system (Sperm Class AnalyzerⓇ, Microptic S.L., Spain) was used to examine sperm motility. Boar spermatozoa were incubated for 30 min and 2 hrs at 37.5℃ with or without TCP. Subsequently, a 2 μL aliquot of the semen sample was placed on a pre-warmed (38℃) Leja counting slide (Leja Products BV, Netherlands), and 10 fields were examined at 37.5℃, assessing a minimum of 500 spermatozoa per sample. The proportion of total motile spermatozoa (%), progressive motile spermatozoa (%), and hyperactive spermatozoa (%) was then determined. Motion kinematic parameters such as the curvilinear velocity (VCL, µm·s-1), straight-line velocity (VSL, µm·s-1), average path velocity (VAP, µm·s-1), and percentage linearity (LIN, %), percentage straightness (STR, %), and wobble percentage (WOB, %) were measured for each spermatozoon.
Assessment of sperm motility
A computer-assisted sperm analysis (CASA) system (Sperm Class AnalyzerⓇ, Microptic S.L., Spain) was used to examine sperm motility. Boar spermatozoa were incubated for 30 min and 2 hrs at 37.5℃ with or without TCP. Subsequently, a 2 μL aliquot of the semen sample was placed on a pre-warmed (38℃) Leja counting slide (Leja Products BV, Netherlands), and 10 fields were examined at 37.5℃, assessing a minimum of 500 spermatozoa per sample. The proportion of total motile spermatozoa (%), progressive motile spermatozoa (%), and hyperactive spermatozoa (%) was then determined. Motion kinematic parameters such as the curvilinear velocity (VCL, µm·s-1), straight-line velocity (VSL, µm·s-1), average path velocity (VAP, µm·s-1), and percentage linearity (LIN, %), percentage straightness (STR, %), and wobble percentage (WOB, %) were measured for each spermatozoon.
Evaluation of sperm viability
Sperm viability was evaluated using the LIVE/DEADTM Sperm Viability Kit (Thermo Fisher Scientific Inc., USA), which comprises the DNA stains SYBR14 (100 nM) and propidium iodide (PI; 10 μM). Initially, the incubated spermatozoa (1 × 108 cells·mL-1) were washed twice with a phosphate-buffered saline solution containing 0.1% (w·v-1) polyvinyl alcohol (PBSPVA). Subsequently, the spermatozoa were stained, and a fluorescence microscope equipped with a camera and imaging software was employed to capture images (Nikon Eclipse Ci microscope, Nikon Instruments Inc., Japan). The spermatozoa were categorized and counted as either viable (SYBR14 positive) or non-viable (PI positive).
Measurement of acrosome integrity
Sperm cells were fixed in a 95% ethanol solution and then kept at a temperature of 4℃ for half an hour. The fixed sperm cells were subsequently dried on the microscope slides and subjected to staining using fluorescein isothiocyanate-labeled Pisum sativum agglutinin (FITC-PSA) at a concentration of 5 μg·mL-1. This staining process lasted for 10 min, during which the FITC-PSA molecules bound to specific constituents of the acrosome. To assess acrosome integrity, the stained samples were examined using a fluorescence microscope equipped with a camera and imaging software (Nikon Eclipse Ci microscope, Nikon Instruments Inc., Japan). The resulting images were classified into two categories based on the fluorescent signals observed in the sperm heads. Sperm cells exhibiting a green fluorescence were categorized as having an intact acrosome, while sperm heads displaying either partial green fluorescence or lacking such fluorescence in the head region were indicative of spermatozoa with damaged or acrosome-reacted states.
Assessment of chromatin stability
An acridine orange assay was carried out following the methodology outlined by the previous study (Martin et al., 2007) with some modifications. Slides with sperm samples were prepared and allowed to air dry. These slides were then fixed using Carnoy’s solution (methanol : glacial acetic acid, 3 : 1) and left to air-dry once more. Following this, the slides were incubated with tampon solution (composed of 80 mmol·L-1 citric acid and 15 mmol·L-1 Na2 HPO4 , pH 2.5) at a temperature of 75℃ for 5 minutes. Subsequently, the slides were stained using acridine orange (0.2 mg·mL-1), the excess stain was removed with water, and coverslips were placed. Finally, the prepared slides were examined using a fluorescence microscope (Nikon Eclipse Ci microscope, Nikon Instruments Inc., Japan). Spermatozoa possessing normal DNA content exhibited a green color, while those with abnormal DNA emitted hues ranging from yellow-green to red.
Measurement of the levels of intracellular reactive oxygen species (ROS)
The sperm cells were subjected to two rounds of washing using a 0.1% PBS-PVA solution. Subsequently, they were exposed to 1 µM of the fluorescent dye 5-(and-6)-carboxy-2′,7′-dichlorodihydro-fluorescein diacetate (carboxy-H2DCFDA, Thermo Fisher Scientific Inc., USA) at 37℃ for 10 min. Following the incubation, the sperm cells underwent two more rounds of washing using a 0.1% PBS solution. Subsequently, the stained sperm cells were carefully mounted in a Vectashield solution (Vector Laboratories, Inc., USA) and then observed utilizing a fluorescence microscope equipped with a camera (Nikon Eclipse Ci microscope, Nikon Instruments Inc., Japan). This setup facilitated the quantification of the intensity of the fluorescence emitted by the stained sperm cells and thereby measured the amount of reactive oxygen species (ROS) production within the cells.
Real-time PCR
After incubation with or without TCP for 30 min, the sperm were washed three times with PBS-PVA. The total RNA was prepared according to the guidelines of the PureLink™ RNA Mini Kit (Thermo Fisher Scientific, USA) with slight modifications. The RNA concentrations were measured using a nanodrop spectrophotometer (DeNovix DS-11FX, DeNovix Inc., USA). Complementary DNA (cDNA) was synthesized from purified RNA using the TOYOBO ReverTra Ace qPCR RT kit (TOYOBO Inc., Japan) according to the manufacturer’s protocol. Quantitative real-time polymerase chain reaction (qRT-PCR) was performed in triplicate with the SYBR™ Premix Ex Taq™ II (Bioneer Corporation, Korea) using the MyGo Pro PCR cycler (Diagnostic Technology Pty Ltd., Australia). The relative expression levels of mRNA from the target genes were compared with that of the endogenous control glyceraldehyde-3-phosphate dehydrogenase (GAPDH). The sequences of the specific primers (Table 1) used to measure the relative expression of the outer dense fiber of sperm tails 2 protein (ODF2), zona pellucida binding protein 2 (ZPBP2), and A-kinase anchor protein 3 and 4 (AKAP3/ AKAP4) were created using the Primer Blast software (National Center for Biotechnology Information, http://www.ncbi.nlm.nih.gov/).
Results and Discussion
To evaluate the effect of TCP on the functions of boar spermatozoa, the motility and motion kinetic parameters of the sperms were evaluated after incubation with TCP (10 - 200 µM) for 30 min and 2 hours at 37℃, (Table 2; Fig. 1). Dosedependent decrements of motility and motion kinematic parameters were observed in the sperm exposed to TCP compared to the controls (without TCP [w/o] and vehicle control of DMSO) at 30 minutes and 2 hours (Fig. 1A and B). When compared to the controls after incubation for 30 min, a significant reduction in sperm motility was observed in spermatozoa exposed to 50 - 200 μM TCP (83.8 - 89.3% controls vs. 49.3 - 72.2% TCP [50 - 200 µM], p < 0.05 and p < 0.001; Fig. 1A). A similar reduction in motility was observed in the spermatozoa exposed to 50 - 200 μM TCP for 2 hours (78.6 - 79.3% controls vs. 24.2 - 39.0% [50 - 200 µM] TCP, p < 0.01 and p < 0.001; Fig. 1B). The percentages of progressive motility (PR), straightness index (STR), curve speed (VCL), linear speed (VSL), and average path velocity (VAP) decreased in spermatozoa incubated with TCP compared to the controls for both periods of incubation (p < 0.05, p < 0.01, and p < 0.001; Table 2).
Fig. 1
Evaluation of sperm motility. Boar spermatozoa were exposed to varying concentrations of 3, 5, 6-trichloro-2-pyridinol (TCP, or controls; without [W/O] TCP and dimethyl sulfoxide [DMSO]) after incubation for 30 minutes (A) and 2 hours (B). Values are expressed as mean ± SEM (standard error of the mean). ** p < 0.01, *** p < 0.001.
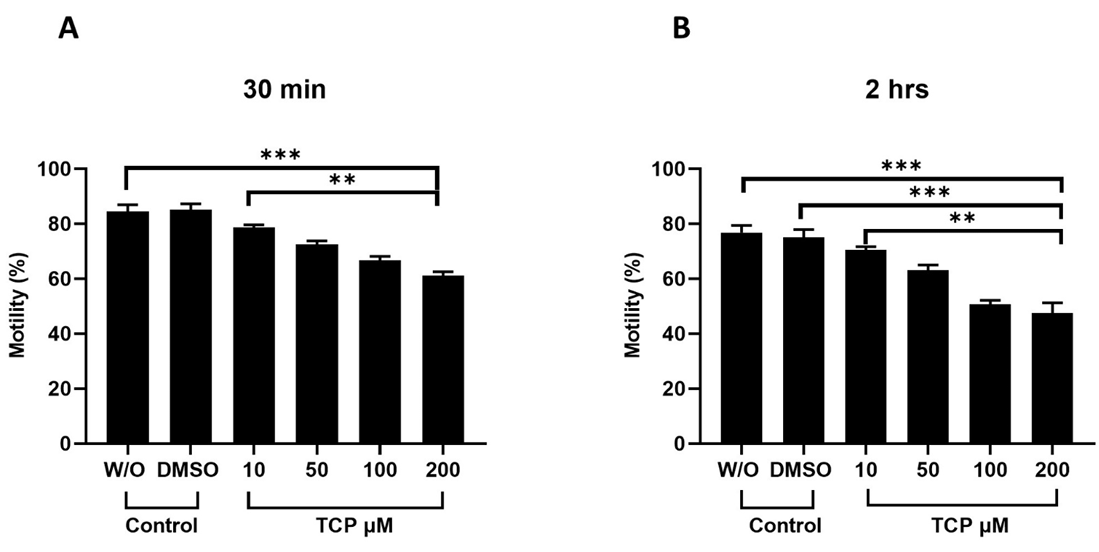
Table 2
Effects of 3, 5, 6-trichloro-2-pyridinol (TCP) on sperm motion kinematics after 30 min and 2 hours of incubation.
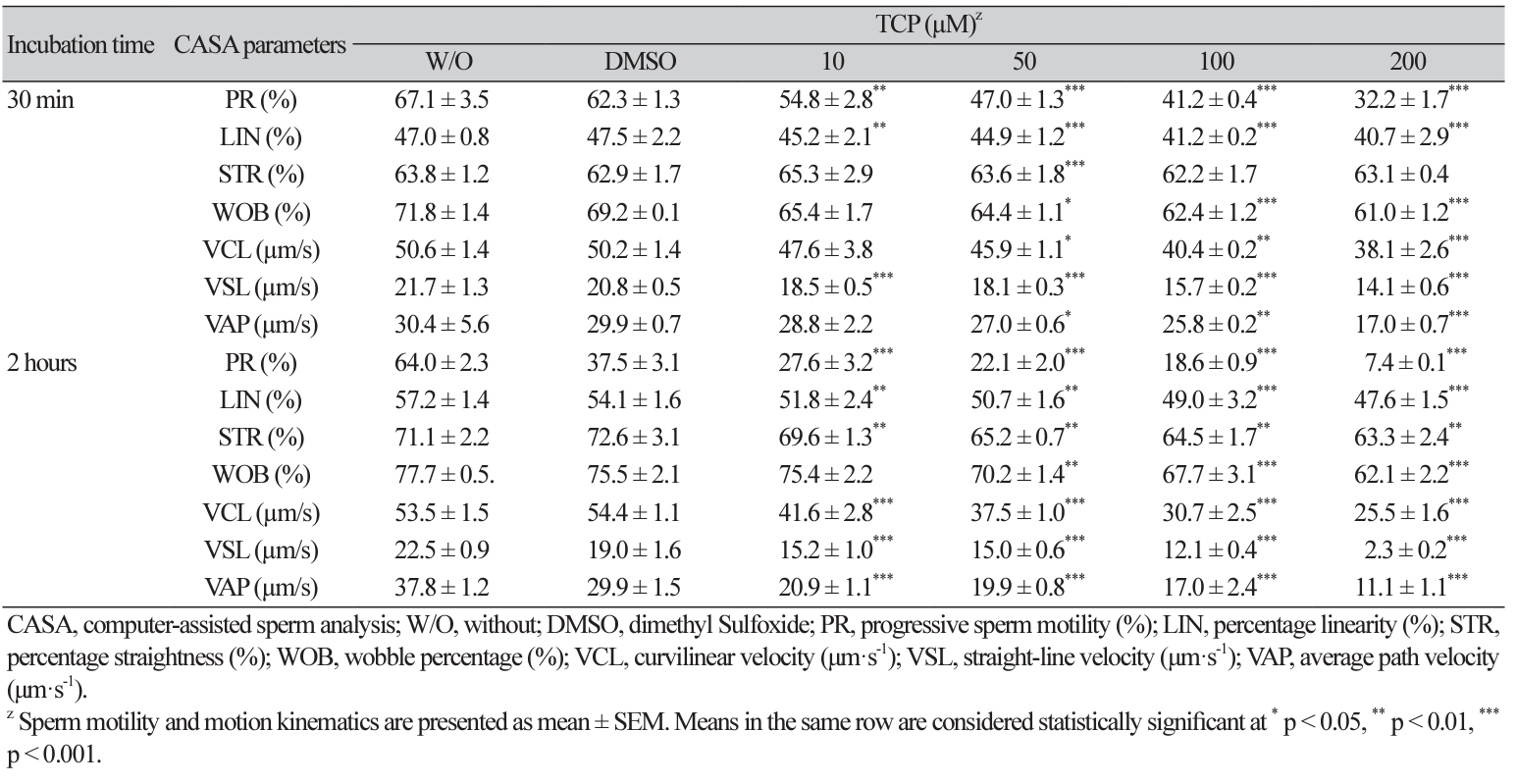
To evaluate viability, sperm samples were stained with SYBR14 and propidium iodide (PI) and images were taken under the fluorescence microscope. Sperms exposed to 10 - 200μM TCP for 30 min and 2 hours, showed a statistically significant dose-dependent decrease in viability compared to control and DMSO across all concentrations and for both incubation periods. As shown in Fig. 2A, the samples incubated with 200 µM TCP for 30 min showed significantly lower viability (30.7 %) compared to the control (80.5 - 81.95%, p < 0.001). Similarly, after 2 hours of incubation, dose-dependent reductions of viable sperms were observed in the TCP-exposed groups compared to controls (78.6 - 79.3% in controls vs. 24.2 - 64.2% in TCP [50 - 200 µM], p < 0.01 and p < 0.001; Fig. 2B).
Acrosome integrity was assessed by staining with FITC-PSA. The lowest intact acrosome percentage was observed in sperm exposed to 200 μM TCP after 30 min of incubation (94.9 - 97.1% in controls vs. 54.5% at 200 μM TCP, p < 0.001; Fig. 3A), and decrement was higher in sperm incubated with 50 - 200 μM TCP for 2 hours (91.0 - 97.0% in controls vs. 36.4 - 64.6% in TCP in 50 - 200 µM TCP, p < 0.05, p < 0.01, and p < 0.001; Fig. 3B).
Fig. 2
Sperm viability was examined after treatment with different concentrations of 3, 5, 6-trichloro-2pyridinol (TCP, or controls; without [W/O] TCP and dimethyl sulfoxide [DMSO]) and incubation period of 30 minutes and 2 hours. Values are expressed as mean ± SEM (standard error of the mean). * p < 0.05, ** p < 0.01, and *** p < 0.001.
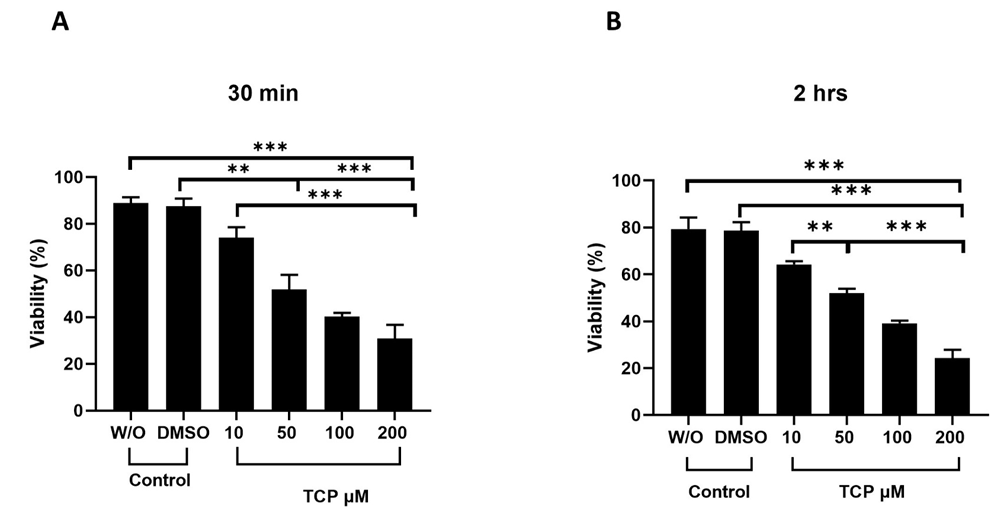
Fig. 3
Acrosome integrity was evaluated in boar sperm exposed to varying concentrations of 3, 5, 6-trichloro-2-pyridinol (TCP or controls; without [W/O] TCP and dimethyl sulfoxide [DMSO]) and two different periods of incubation, 30 minutes and 2 hours. Values are expressed as mean ± SEM (standard error of the mean). ** p < 0.05, *** p < 0.001.
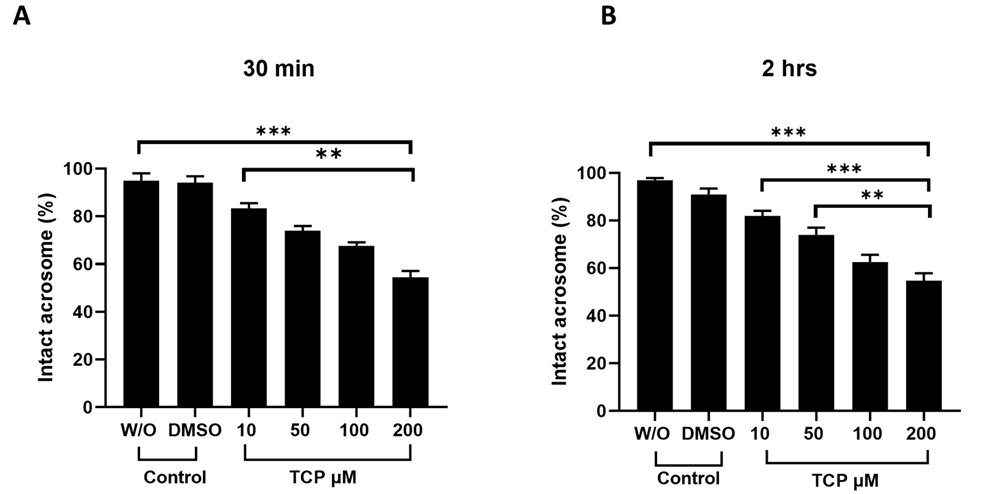
To examine chromatin stability, sperm samples were stained with acridine orange and observed under a fluorescence microscope. Compared to the controls, the rate of normal chromatin significantly decreased with the increase in the TCP concentrations after 30 min incubation (95.1 - 95.5% in controls vs. 47.7% at 200 µM TCP, p < 0.001; Fig. 4A) and similarly after 2 hours of incubation (92.8 - 94.0% in the controls vs. 24.5 - 84.5% in 10 - 200 µM TCP, p < 0.05 and p < 0.001; Fig. 4B).
Fig. 4
Chromatin stability of spermatozoa exposed to varying concentrations of 3, 5, 6-trichloro-2pyridinol (TCP or controls; without [W/O] TCP and dimethyl sulfoxide [DMSO]) and two different periods of incubation, 30 minutes (A) and 2 hours (B). Values are expressed as mean ± SEM (standard error of the mean). The different superscripts in each group denote a significant difference at ** p < 0.01, *** p < 0.001.
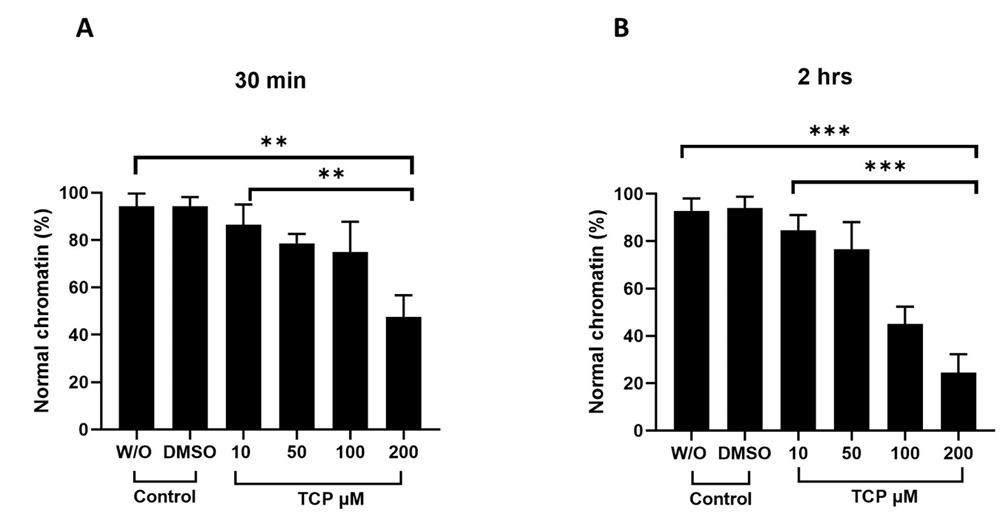
To evaluate the intracellular ROS production, spermatozoa incubated in the absence or presence of TCP were stained with carboxy-2′,7′-dichlorofluorescein diacetate solution at 37℃ for 10 min, and the fluorescence intensity was measured in stained samples under the fluorescence microscope. Higher fluorescence intensities were obtained in sperm treated with 10 - 200 μM TCP compared to the control groups (Fig. 5A). After 30 min of incubation a gradual dose-dependent increase in the f luorescence intensity for ROS was observed, at concentrations ranging from 10 to 200 μM (p < 0.01 and p < 0.001; Fig. 5A). Similar increases were observed in the sperm exposed to TCP for 2 hrs (p < 0.01 and p < 0.001; Fig. 5B). For both periods of incubation, the generation of ROS was the highest in spermatozoa incubated with 200 μM TCP.
Fig. 5
Production of reactive oxygen species (ROS) in spermatozoa exposed to varying concentrations of 3, 5, 6-trichloro-2-pyridinol (TCP or controls; without [W/O] TCP and dimethyl sulfoxide [DMSO]) and different periods of incubation, 30 minutes and 2 hours. Values are expressed as mean ± SEM (standard error of the mean). ** p < 0.01, *** p < 0.001.
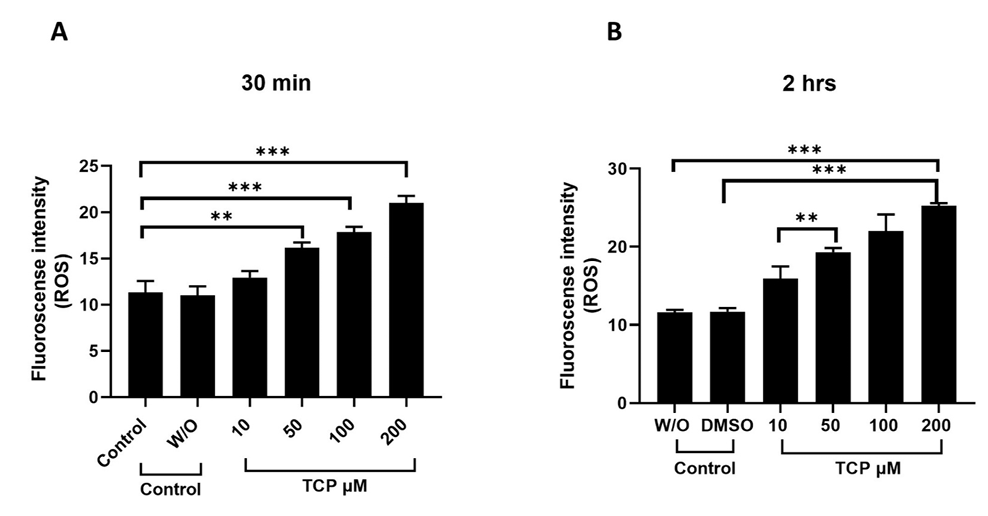
The relative mRNA expressions of ODF2, ZPBP2, AKAP3 and AKAP4 associated with the fertilizing capacity of spermatozoa were examined in the sperm incubated with TCP (Fig. 6). The expression of ODF2, AKAP3 and AKAP4 were down-regulated significantly in the sperm exposed to TCP compared to the controls (p < 0.05 and p < 0.01; Fig. 6A, C, and D).
Fig. 6
Relative mRNA expression of outer dense fiber sperm tails protein 2 (ODF2; A), zona pellucida binding protein 2 (ZPBP2; B), and A-kinase anchor protein 3 (AKAP3; C), and A-kinase anchor protein 4 (AKAP4; D) by quantitative real-time PCR in spermatozoa exposed to varying concentrations of 3, 5, 6-trichloro-2-pyridinol (TCP or controls; without TCP [W/O] and dimethyl sulfoxide [DMSO]). Relative expression levels of mRNA from the target genes were compared with that of the endogenous control glyceraldehyde-3-phosphate dehydrogenase (GAPDH). Values are expressed as mean ± SEM (standard error of the mean). * p < 0.05, ** p < 0.01.
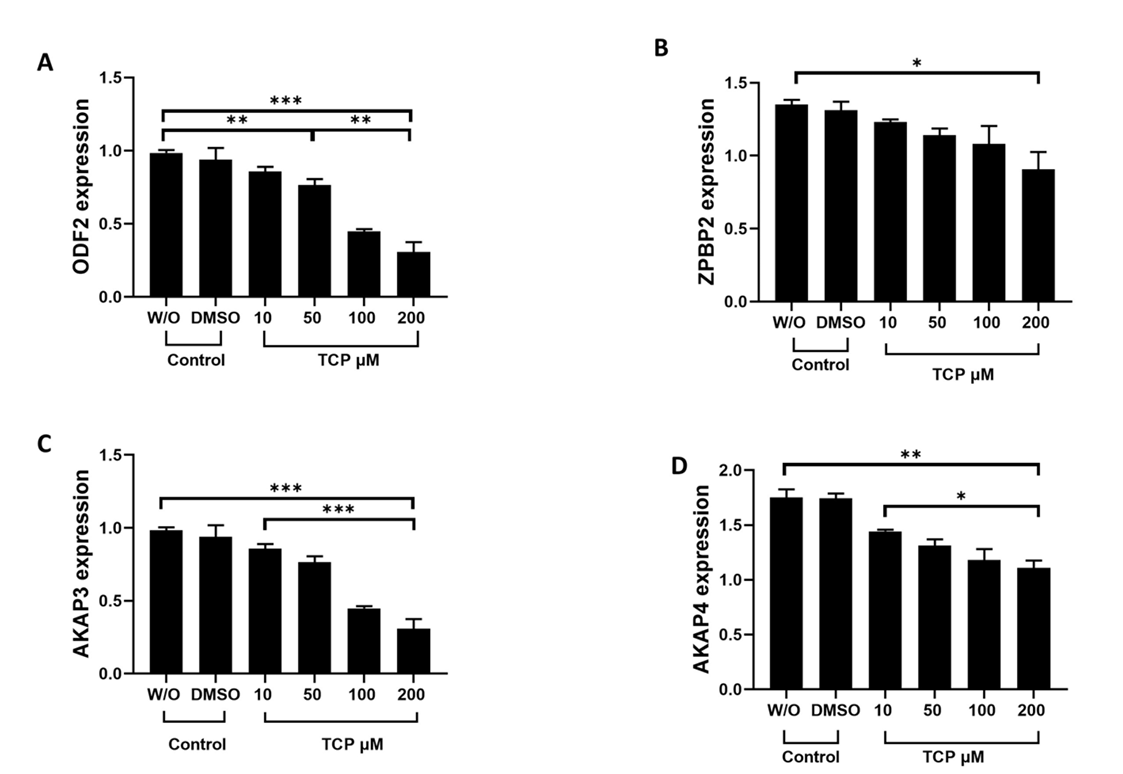
This study aimed to investigate the impact of acute exposure to TCP on the viability and fertilizing ability of boar spermatozoa. OPs and their metabolites have long been recognized for their persistence in the environment and their ability to interact with the physiological mechanisms of various organisms leading to widespread health disorders (Giesy et al., 2014; Sidhu et al., 2019). The effects of exposure to these pesticide metabolites not only influence the metabolic, physiological, and reproductive health of animals but also pose risks to future generations (Gavrilescu et al., 2015). TCP was recognized as a significant biomarker and one of the ultimate byproducts of CPF, frequently found in urine and blood (Fortenberry et al., 2012; Condette et al., 2014). CPF can enter the animal body by ingestion, inhalation, or penetrating the epidermis, then tissues, organs, milk, and blood circulation (Kim et al., 2013; Wołejko et al., 2022). After entry into the body, CPF first undergoes cytochrome P450-dependent oxidative desulfuration and converts to chlorpyrifos-oxon and then is hydrolyzed by A-esterase to produce diethylphosphate (DEP) and TCP (Foxenberg et al., 2007; Condette et al., 2014). Previous studies revealed the cytotoxicity of CPF and TCP in the male reproductive system, and there is emerging evidence regarding the harmful effects of CPF metabolites (Ellison et al., 2011). In a recent study, primary Sertoli cells were exposed to varying concentrations of TCP, ranging from 0.1 to 1,000 µM, and the results showed that TCP caused a reduction in the viability of primary Sertoli cells, and this reduction was directly proportional to the TCP concentration (Gao et al., 2021).
OPs have been observed to interfere with the cellular and mitochondrial systems responsible for antioxidant defense (Binukumar et al., 2010). They can elevate the production of ROS (Abdollahi et al., 2004) and disrupt the uptake of calcium ions (Karami-Mohajeri and Abdollahi, 2013). These actions contribute to oxidative stress and damage, potentially leading to harmful effects on cellular components and DNA. Additionally, the disruption caused by these pesticides can initiate pathways that prompt cell death (Karami-Mohajeri and Abdollahi, 2013). Adenosine triphosphate (ATP) is essential for many sperm functions such as sperm motility, and cell metabolism including fusion events associated with fertilization (Jeyendran et al., 2021). Because of the crucial role of mitochondria in ATP production, ROS generation, and integration of steroid receptor functions, any dysregulation of their function could affect sperm motility and fertilization ability (Anifandis et al., 2018). Exposure to OPs like CPF was observed to result in a decrease in sperm motility and male fertility in mice by impairing mitochondrial function and increasing ROS levels (Zhang et al., 2020). Similarly in our study, we observed a dosedependent increase in ROS production and a decrease in motility in sperm samples exposed to TCP.
Our results show that the exposure of sperm samples to TCP contributes to alterations in the chromatin integrity in a dosedependent manner. OPs are involved in the phosphorylation of proteins, altering their function and interaction with other proteins. Changes in the sperm nuclear proteins (protamine), which are responsible for packaging DNA during the final stages of spermatogenesis, contribute to the development of male reproductive toxicity (Piña-Guzmán et al., 2005).
Differential expression patterns of specific families or types of sperm proteins following their exposure to pesticides have been investigated to understand how this process affects sperm viability and function. The structural integrity of the sperm flagella is crucial for sperm motility. ODFs are a component of the complex structure surrounding the central axoneme within the flagellum (Zhao et al., 2018). Decreases in the protein expression of ODF2 in the sperm samples exposed to TCP are positively correlated with a decline in sperm motility which is suggestive of structural alterations in the sperm flagella. ZPBP2 is highly expressed in sperm cells and is required for the process of sperm maturation (Lin et al., 2007). During fertilization, ZPBP2 binds to the zona pellucida, which surrounds the oocyte to allow sperm penetration (Kozlovsky and Gefen, 2013). Therefore, a lower expression of the ZPBP could result in the reduced ability of the spermatozoa to bind to the zona pellucida thereby decreasing the possibility of a successful fertilization. AKAP4 is an essential component of sperm biology, particularly in relation to the flagellar structure, sperm motility, and potentially other aspects of sperm function like chemotaxis and capacitation (Blommaert et al., 2019). It has been shown that the absence or weak expression of AKAP4 is associated with poor sperm motility and dysplasia of the fibrous sheath (DFS) in male mice (Miki et al., 2002). Similarly, it was reported that the lack of AKAP3 could lead to disruptions in the integrity of the subcellular structure and proteome of mouse sperm and cause male sterility (Xu et al., 2020). Therefore, an analysis of the expression patterns of different types of sperm proteins indicates that direct exposure of the sperm to TCP can lead to lower sperm motility and fertilization ability, potentially due to differences in the structural characteristics of the sperm proteins.
The maturation of spermatogonia to spermatozoa is a complex sequence of events involving multiple stages of cell division and differentiation. Some epidemiological studies have suggested that TCP decreases human sperm motility by affecting semen quality including an increased DNA fragmentation index and decreased testosterone levels (Dziewirska et al., 2019; Gao et al., 2021). By conducting this study, we sought to deepen our understanding of how acute exposure to TCP affects boar spermatozoa, adding to the growing body of evidence regarding the reproductive toxicity of pesticide metabolites and their potential implications for both animal and human health.
Conclusion
Exposure to TCP, a metabolite of chlorpyrifos reduces the motility, motion kinematics, viability, and acrosomal integrity of boar spermatozoa while increasing the intracellular ROS production. Also, the relative mRNA expressions of ODF2, ZPBP2, AKAP4, and AKAP3 were downregulated in sperm exposed to TCP. All these results indicate that the direct exposure of spermatozoa to TCP interferes with male reproductive functions and leads to decreased fertilization.
Acknowledgments
We would like to thank Professor Stephen A Krawetz of the Department of Obstetrics and Gynecology, Center for Molecular Medicine and Genetics, Wayne State University School of Medicine, USA for his help with boar sperm mRNA preparation. This work was supported by a Research Promotion Program of SCNU.