Introduction
The CRISPR/Cas system is derived from a bacterial adaptive immune system acquired by prokaryotic species when a viral infection occurs (Jansen et al., 2002; Mojica et al., 2005; Pourcel et al., 2005). An infected host cell retains a 21 - 37 nucleotide foreign DNA fragment from invaders and coalesces the fragment into its CRISPR locus (Mojica et al., 2005; Pourcel et al., 2005). When invaders with the corresponding protospacer (Barrangou et al., 2007) infect the host cells, pre-CRISPR RNAs (pre-crRNAs) are transcribed along with trans-activating CRISPR RNAs (tracrRNAs) that are partially complementary to the crRNA (Brouns et al., 2008; Marraffini and Sontheimer, 2008). Together, crRNAs and tracrRNAs form an RNA duplex that acts as a guide RNA (gRNA) and the association of Cas9 nuclease completes the development of the crRNA-Cas9 complex (Hale et al., 2008; Hale et al., 2009). Directed by the gRNA, Cas9 recognizes target sequences directly upstream of protospacer adjacent motif (PAM) (3′-NGG) and silences invading exogenous nucleic acids by generating a double-strand break (DSB) 3 bp upstream of PAM (Mojica et al., 2009). Using DNA repair mechanisms such as non-homologous end joining (NHEJ) and homology direct repair (HDR) in the presence of template DNA, DSBs can be mended (Babu et al., 2011). NHEJ occurs more often than HDR and its error-prone property of repair introduces insertion or deletion mutations (InDel mutations) at the target site of the gene of interest (Lieber, 2010) (Fig. 1).
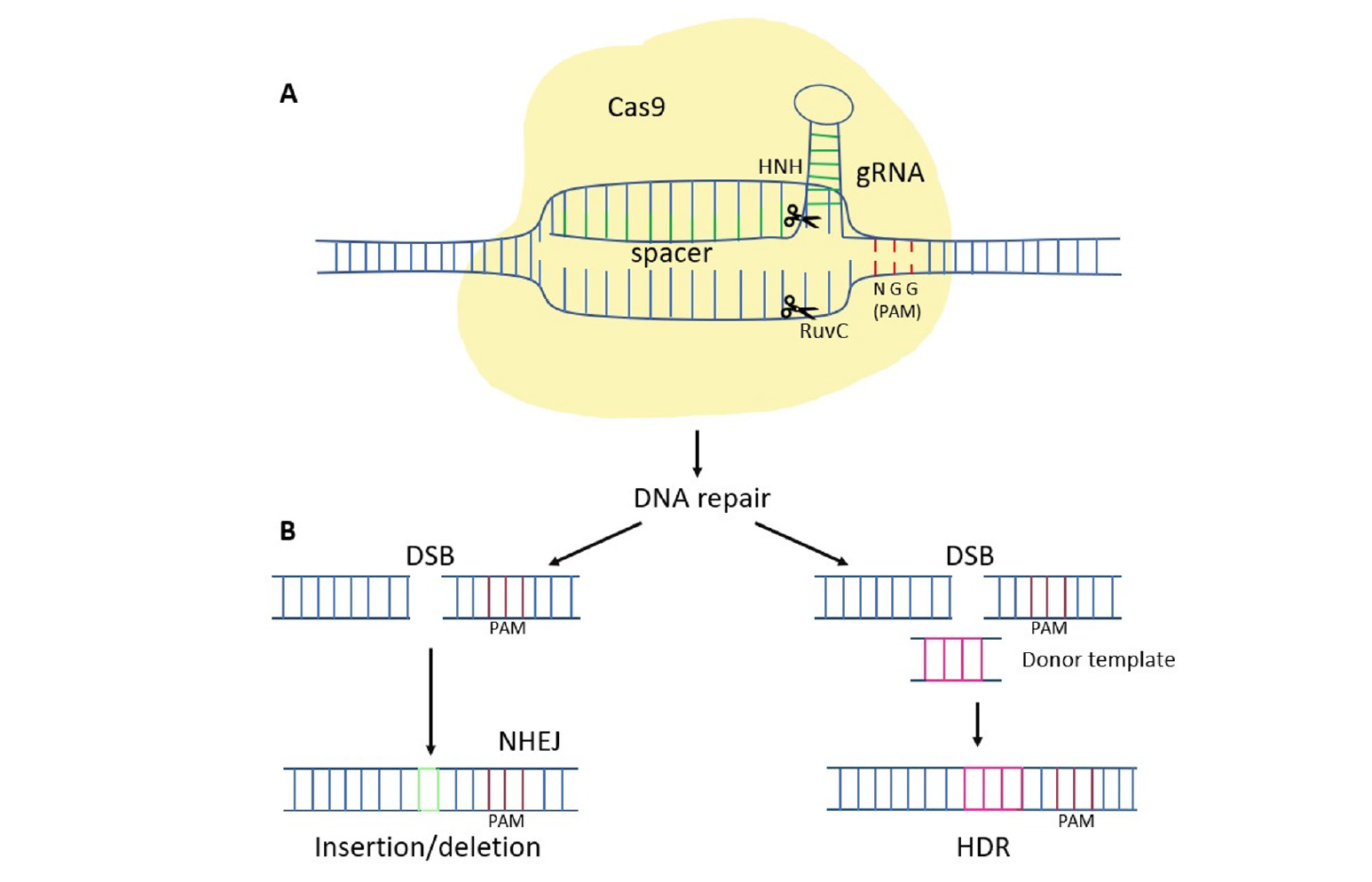
Fig. 1. Scheme of the clustered regularly interspaced short palindromic repeats/CRISPR-associated protein 9 (CRISPR/Cas9) system and two prominent DNA repair mechanisms after the double-strand break (DSB) generated by Cas9 endonuclease activity. (A) Structure of the Cas9 + single guide RNA (sgRNA) complex. (B) DSBs can be repaired either by non-homologous end joining or homology directed repair in the presence of the donor template. HNH, histidine-asparagine-histidine endonuclease; RuvC, RuvC endonuclease; NHEJ, non-homologous end joining; HDR, homology-directed repair; PAM, protospacer-adjacent motif.
In 2012, Doudna and her research fellows first proposed the use of the CRISPR/Cas9 system as a genome editing tool (Jinek et al., 2012), and since then, this revolutionary work has been demonstrated to be practical and powerful over site-directed mutagenesis by numerous research groups (Friedland et al., 2013; Ran et al., 2013). Other variants based on the CRISPR system are also designed to target specific sites of the gene by utilizing Cas9 as a vehicle that delivers companion nucleases and expression regulators to control the viability and expression of the target gene. The CRISPR/Cas9 system is widely used in the genome editing of kingdom plantae (Belhaj et al., 2015; Bortesi and Fischer, 2015) and the use of the CRISPR/Cas system may enable researchers to evade genetically modified organisms-related regulations depending on delivery methods, such as direct delivery of purified Cas9 and sgRNA (ribonucleoprotein, RNP) through polyethylene glycol (PEG) and electroporation (Woo et al., 2015). As the PEG-mediated genome edited Petunia hybrida produced by the research group of Geung-Joo Lee has been approved as a non-genetically modified organism by the ISAAA (2020), the CRISPR/Cas9 system could potentially shorten the process of breeding, under conditions that optimize protocols for regeneration of the target species from the protoplasts readily prepared.
Despite the immense potential of the CRISPR/Cas system, publications on the application of the tool to floricultural crops are rather scarce owing to limited availability of genetic information and polyploidy of ornamental species (Braatz et al., 2017; Kishi-Kaboshi et al., 2018). Especially in ornamental cultivars, color patterns, shapes, longevity of flowering organs, and fragrance are the most demanded and pronounced traits (Noman et al., 2017; Manzoor et al., 2019) to consider targeting because these species are grown in gardens and indoors for their decorative value (Saint-Oyant et al., 2018). The qualitative changes in these features are often related to polyploidy (Manzoor et al., 2019). To overcome these deterring factors, abundant genetic data of diverse species must be built and enhancement of accuracy and efficacy of genome editing tools is essential.
In this paper, we briefly introduce CRISPR/Cas derived genome editing techniques, their application in ornamental species exhibiting decorative features, and future prospects of employing editing tools in floricultural crops.
CRISPR/Cas9 variants
Cas12a (CPF1)
Cas12a is a subtype of the Cas protein and the name is initially originated from the bacterial species Prevotella sp. and Francisella novicida (Cpf1) (Zetsche et al., 2015). Cas12a can be alternatively used after T-rich PAM (5′-NTT) (Zetsche et al., 2015) followed by target sequences. Compared to Cas9, Cas12a can generate DSB 20 nucleotides downstream of the PAM with sticky ends that leave a few nucleotides overhanging its end (Zetsche et al., 2015). Minimum requirements for degrading the target gene make Cas12 shorter in sequences and simpler in structure than Cas9. Cas12a only requires the crRNA and RuvC domain to cleave the non-target strand, while the Nuc domain residing in RuvC cleaves the target strand to generate DSBs (Zetsche et al., 2015; Zetsche et al., 2017) (Fig. 2). In contrast, Cas9 requires an additional HNH domain to cleave the target strand, thereby inducing the DSB (Deltcheva et al., 2011; Chylinski et al., 2013). Cas12a is also more likely to induce mutations at the target site, whereas close proximity of the Cas9 cleavage site to PAM can eventually lead to deletion of PAM, further eliminating the possibility for Cas9 to re-target the specified site (Zetsche et al., 2015). Cas12a has been shown to exhibit on-target effects similar to those of Cas9, while the off-target effects are less than those of Cas9 (Kim et al., 2016; Kleinstiver et al., 2016).
Versatile use of catalytically impaired Cas9
Cas9 has two distinct cleavage domains to generate a DSB. One is HNH for target strand cleavage and the other is RuvC for non-target strand cleavage (Deltcheva et al., 2011; Chylinski et al., 2013). Mutations in these key domains impair the endonuclease activity of Cas9 (dCas9); however, dCas9 remains capable of binding to the target DNA sequences (Fujita and Fujii, 2013; Qi et al., 2013). The mobility of dCas9 has drawn the attention of researchers, and various applications have been recognized.
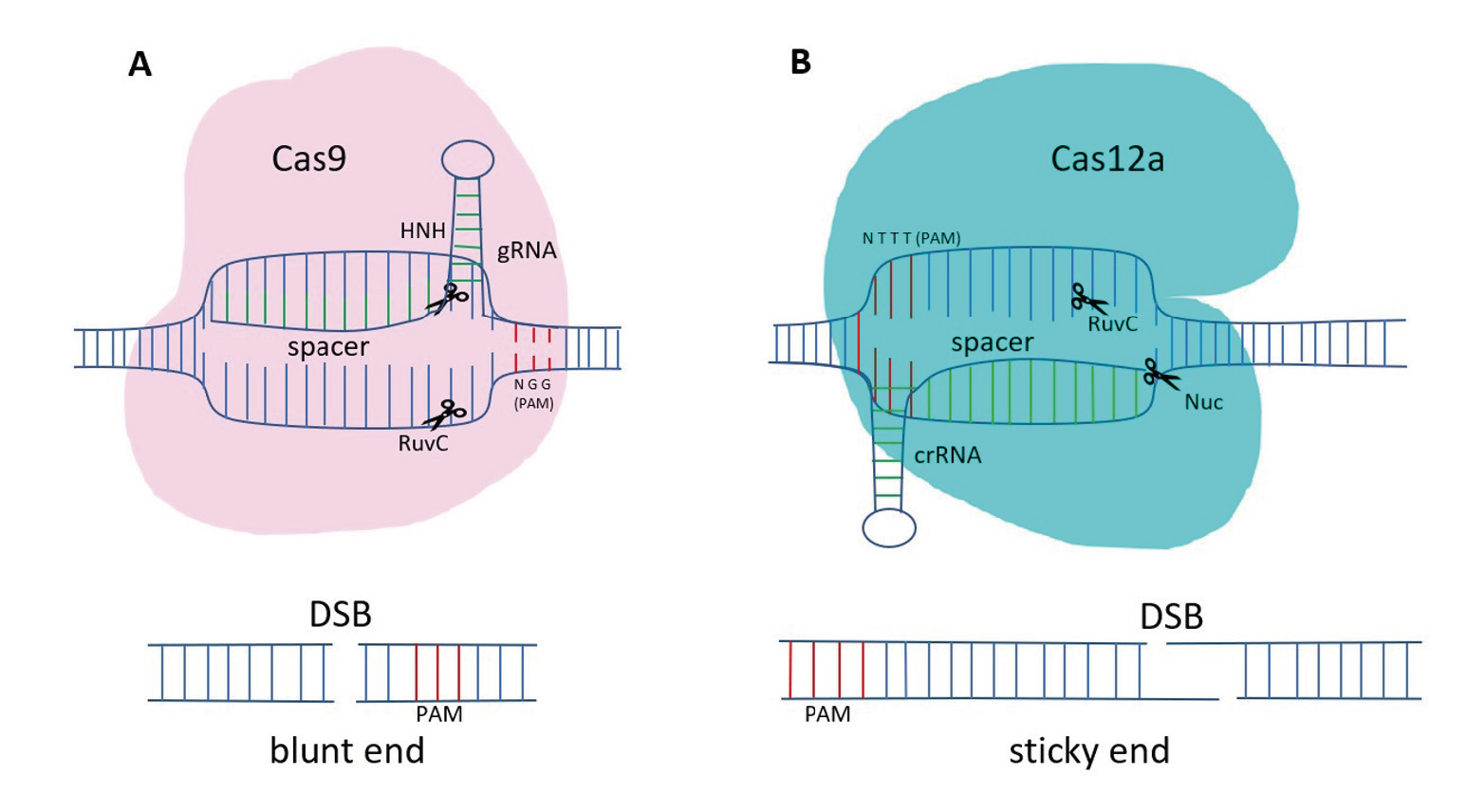
Fig. 2. Differences between CRISPR-associated protein 9 (Cas9) and CRISPR-associated protein 12a. (A) Typical Cas9 from Streptococcus pyogenes generates a blunt-ended double-strand break (DSB) 3 bp upstream of the protospacer-adjacent motif (PAM). (B) DSB caused by Cas12a is located 20 bp downstream of the putative PAM. HNH, histidine-asparagine-histidine endonuclease; RuvC, RuvC endonuclease; gRNA, guide RNA; crRNA, CRISPR RNA; Nuc, nuclease.
dCas9-FokI
The employment of catalytically inactive dCas9 with FokI nuclease has been proposed to reduce off-target editing of Cas9 (Guilinger et al., 2014; Hara et al., 2015). dCas9 fused to FokI detects the target site using gRNA, and the cleavage event is conducted by FokI (Fig. 3). A dCas9-FokI monomer alone cannot generate DSB, and therefore, requires another set of dCas9-FokI targeting nearby sequences. dCas9-FokI can be applied to target repetitive DNA (Guilinger et al., 2014; Hara et al., 2015; Wyvekens et al., 2015).
Base editing
Base editing is devised to induce a point mutation at the target site of DNA or RNA, thereby altering a base to another desired base without generating DSBs (Rees and Liu, 2018; Kim et al., 2019). Together with various base editors, this approach also employs dCas9 or Cas9 nickase (Cas9n), which is only capable of introducing a single strand break because of the mutation in one or the other cleavage domain. The conversion of bases is limited to that of C to T, G to A, A to G, and T to C because of the lack of proper known deaminases (Komor et al., 2016; Nishida et al., 2016; Gaudelli et al., 2017; Vogel et al., 2018). Because detrimental mutations largely take the point mutation into account (Landrum et al., 2014; Landrum et al., 2016), considerable efforts have been made to seek means for reverting the mutation to its original state.
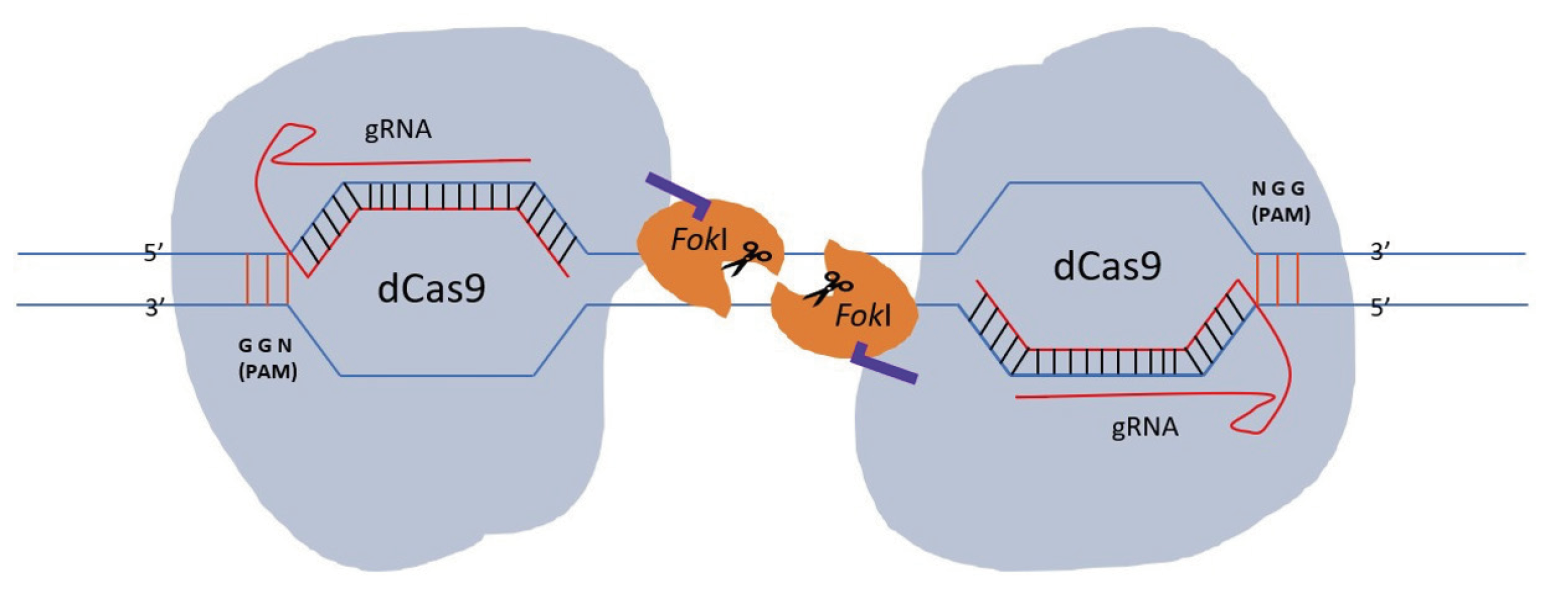
Fig. 3. Scheme of dead Cas9-FokI (dCas9-FokI). Two FokI enzymes attached by dCas9 replace the catalytic activity of Cas9. The system requires two molecules of dCas9 each bound to the flanking strand of the target region to generate a double-strand break with enhanced precision. gRNA, guide RNA; PAM, protospaceradjacent motif.
CRISPRi
Utilization of dCas9 is also able to suppress gene expression (CRISPRi) (Qi et al., 2013). Knockdown of a gene of interest is achieved by the binding activity of dCas9 to the promoter of the gene and base pairing of gRNA complementary to the non-template strand, consequently resulting in transcriptional repression of the gene by RNA polymerase blockage (Larson et al., 2013; Qi et al., 2013). Initially, CRISPRi comprised dCas9 and chimeric gRNA comprised guiding sequences, a Cas9-binding hairpin, and transcription terminator attained from Streptococcus pyogenes (Larson et al., 2013; Qi et al., 2013) (Fig. 4). Attempts to widen the range of CRISPRi applications have made use of repressing elements such as KRAB (Gilbert et al., 2013) and MeCP2 (Xiong et al., 2019). In plants, Piatek et al. (2015) demonstrated that dCas9 alone and dCas9-SRDX efficiently downregulated PDS in Nicotiana benthamiana.
Although one may associate RNA interference (RNAi) with CRISPRi, the CRISPRi complex shows high specificity for the target site and exceptionally low rates of off-target effects and is able to modulate repression of multiple targets, whereas the use of RNAi can lead to unintended downregulation of genes with similar sequences (Smith et al., 2017).
CRISPRa
Using a similar approach as that of CRISPRi, attaching effector domains with upregulating properties to dCas9 can activate the expression of the target gene (CRISPRa) (Gilbert et al., 2013). Transcriptional activators such as VP64 (Maeder et al., 2013; Nihongaki et al., 2015) and p65 (Nihongaki et al., 2015) are commonly used for CRISPRa, and fusion of supplementary activator domains increases its activating proficiency (Fig. 4). A framework for applying CRISPRa in plantae employed EDLL and TAD-AD transcription factors to upregulate the NbPDS gene (Piatek et al., 2015).
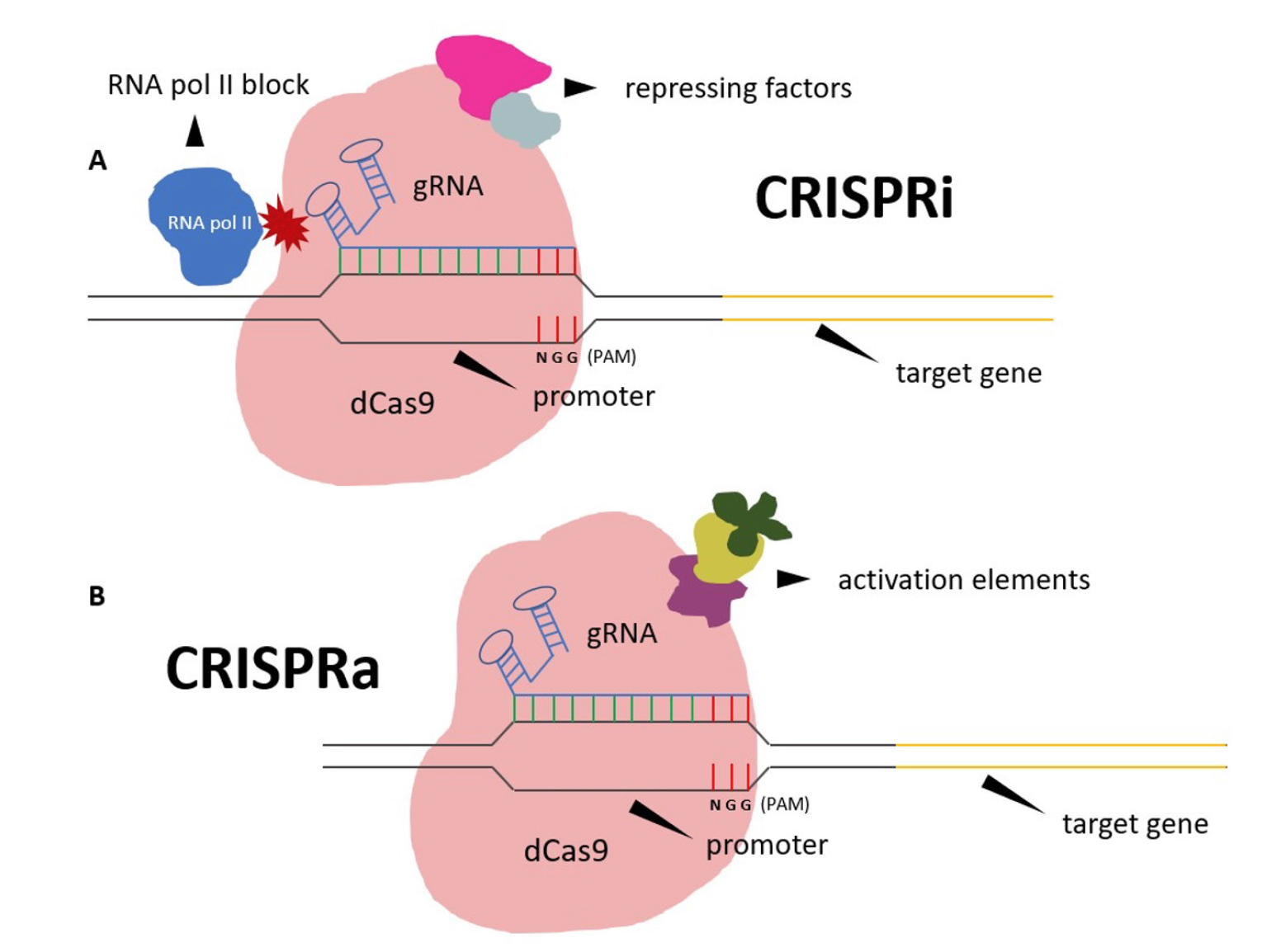
Fig. 4. Composition of CRISPR interference (CRISPRi) and CRISPR actiation (CRISPRa). (A) Repressing factors down-regulate the target gene expression by blocking RNA polymerase II activity. (B) Attachment of activation elements upregulates the target gene expression by amplified promoter activity. gRNA, guide RNA; dCas9, dead Cas9; PAM, protospacer-adjacent motif.
Prime editing
Prime editing is the latest development in the CRISPR/Cas chronicle. Its intricacy and precision in editing undesired bases facilitates all 12 possible bases to base conversions, overcoming the limitations of existing base editors (Anzalone et al., 2019). In combination with Cas9 H840A (nCas9 with deactivated HNH domain) and its C-terminal fused with modified Moloney murine leukemia virus reverse transcriptase (M-MLV-RT) and prime editing guide RNA [pegRNA comprising the primer-binding site, reverse transcriptase (RT) template with edit sequences, and sgRNAs], the prime editing system works to reshape the target site (Anzalone et al., 2019) (Fig. 5).
Precision editing via the prime editor is achieved by the following steps: nCas9 binds to the target site under the direction of sgRNA and nicks the non-target stand. The nicked strand hybridizes to the primer-binding site and reverse transcription extends the edited strand by making use of the polymerized strand as a primer. The 3′ flap containing the edit and the unedited 5′ flap undergo flap equilibration, resulting in edited DNA by subsequent activities of structure-specific endonucleases and 5′ exonucleases (Anzalone et al., 2019; Marzec et al., 2020).
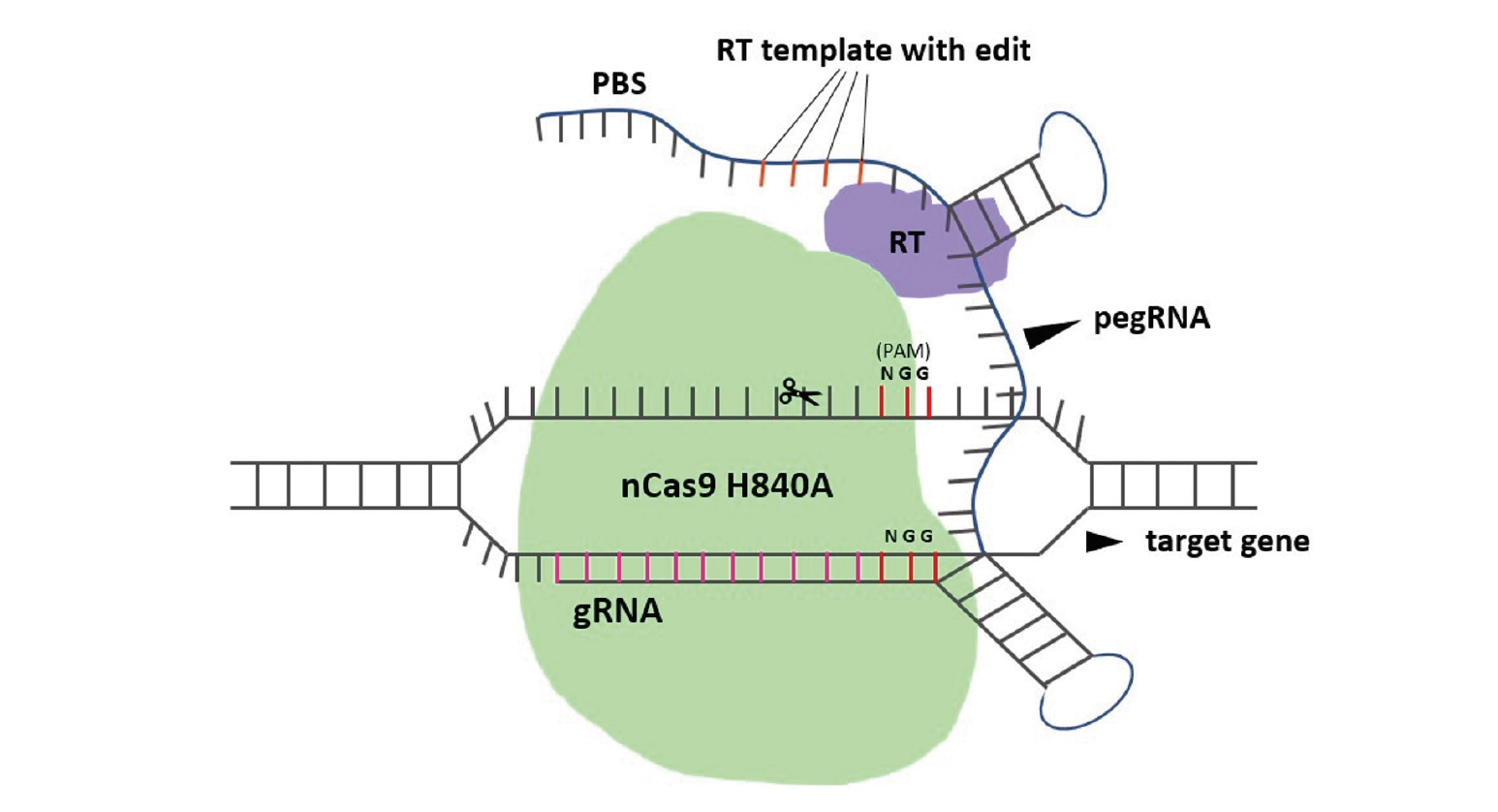
Fig. 5. Structure of prime editor 3. Cas9 nickase (H840A Cas9) generates a single-strand break on the nontarget strand and the nicked strand directly polymerizes with a short-edited strand produced by reverse transcriptase attached on Cas9 nickase. The modified sequences of the reverse transcriptase (RT) template are part of prime editing guide RNA (pegRNA). PBS, primer binding site; PAM, protospacer-adjacent motif.
The initial research produced generations of the prime editor revised up to prime editor 3 (Anzalone et al., 2019). Lin et al. (2020) instantly applied the system in rice and wheat for validation, and plant prime editor 3 has been proven to be adoptable in higher plants (Li et al., 2020).
CRISPR Knock-in
In the presence of a donor template, the DSB can be mended by HDR (Liang et al., 1998; Lieber, 2010). Operation of HDR with a manipulated donor template containing viable sequences allows foreign DNA fragments to “Knock-in” (Zheng et al., 2014) while repeated generation of the DSB on the target sequences by Cas9 routinely “Knock-outs” the gene through NHEJ (Wu et al., 2013). Employing CRISPR knock-in permits the performance of a wide range of genetic engineering from base substitution to removal of unwanted sequences for gene replacement and insertion (Schaeffer and Nakata, 2015), depending on the length of the modified sequences within homology arms (Gilles et al., 2015; Yu et al., 2017; Roth et al., 2018) and the purpose of the experiment. An ideal donor template consists of a 5′ homology arm (left arm) and 3′ homology arm (right arm) flanking replacing sequences, and each arm must share identical sequences to the host gene for homology recognition (Zheng et al., 2014) (Fig. 6). For the nested gene to properly function in the host gene, a promoter needs to be accompanied at the end of the left arm (Li et al., 2016). Double strand DNA (dsDNA) and single strand DNA (ssDNA) can be used as the donor template; however, recent studies have shown that the use of ssDNAs as templates manifested higher on-target integration rates and lower cytotoxicity than those with dsDNA donors (Roth et al., 2018; Li et al., 2019). Successful operation of the CRISPR knock-in has been demonstrated in rice (Zhang et al., 2016; Xu et al., 2020b) and tomato (Yu et al., 2017).
Application of CRISPR/Cas for floricultural crops
Initial studies of CRISPR/Cas9-mediated transformation in ornamental species mainly targeted genes related to chlorophyll synthesis and nitrogen accumulation, such as phytoene desaturase (Yan et al., 2019; Zhu et al., 2019) and nitrate reductase (Subburaj et al., 2016) to induce a conspicuous phenotype, albinism and yellowing, as the transformed plant material would immediately show a sign of the mutations. Subburaj et al. (2016) applied PEG-mediated delivery of the RNP complex to produce mutant petunia protoplasts free of external DNA (Table 1).
Later studies focused on modification of flower colors and successfully altered flower colors by targeting genes involved in anthocyanin biosynthesis (Watanabe et al., 2017; Nishihara et al., 2018; Watanabe et al., 2018; Yu et al., 2020). Among these experiments, Watanabe et al. (2017) had a distinct approach to increase carotenoid content in petals of Japanese morning glory (Ipomoea nil) by targeting carotenoid cleavage dioxygenase 4 (CCD4), which is known to reduce carotenoid accumulation. A DNA-free approach was applied to obtain a complete modification of flower color by simultaneous targeting of both F3HA and F3HB loci via CRISPR/Cas9 ribonucleoproteins in petunia (Yu et al., 2020).
Recently, the primary focus of genome editing in ornamental species has moved to extending the vase life of reproductive organs (Shibuya et al., 2018; Xu et al., 2020a). Shibuya et al. (2018) identified a Nac transcription factor Ephemeral 1, whose expression is predominant in petals of Japanese morning glory. Knockout of InEPH1 resulted in delayed petal senescence in Japanese morning glory. In 2019, prolonged flower longevity was observed in petunia by modulating ethylene biosynthesis (Xu et al., 2020a).
Despite the ever-increasing fame of CRISPR/Cas9, publications regarding the improvement in ornamental value in floricultural crops are barely noticeable owing to the factors described in chapter 3.
Factors impeding the application of CRISPR/Cas9 for ornamental species
Diversity could be said to be a key attribute of ornamental species (Clark et al., 2003). Numerous ornamental species are introduced to the floriculture industry each year (Adkins et al., 2003). Because thorough considerations must be made to determine the target gene and an elucidated biosynthetic pathway is required prior to genome editing, the diversity of floricultural crops further complicates the application of the CRISPR/Cas9 system. Currently, the majority of genome editing work in plantae is focused on model plants and horticultural crops (Karkute et al., 2017) (Fig. 7) because of easier access to genetic information and facilitated protocols for cultivation than those in floricultural crops. The most frequently used plant species for CRISPR-mediated genome editing since 2013 is Asian rice (Oryza sativa, 217 publications). Thale cress (Arabidopsis thaliana, 104), tomato (Solanum lycopersicum, 89), common wheat (Triticum aestivum, 66), soybean (Glycine max, 36), potato (Solanum tuberosum, 32), and maize (Zea mays, 28) are next in descending order. Adequate genetic studies for each species need to be conducted to promote the use of the CRISPR system for floricultural crops.
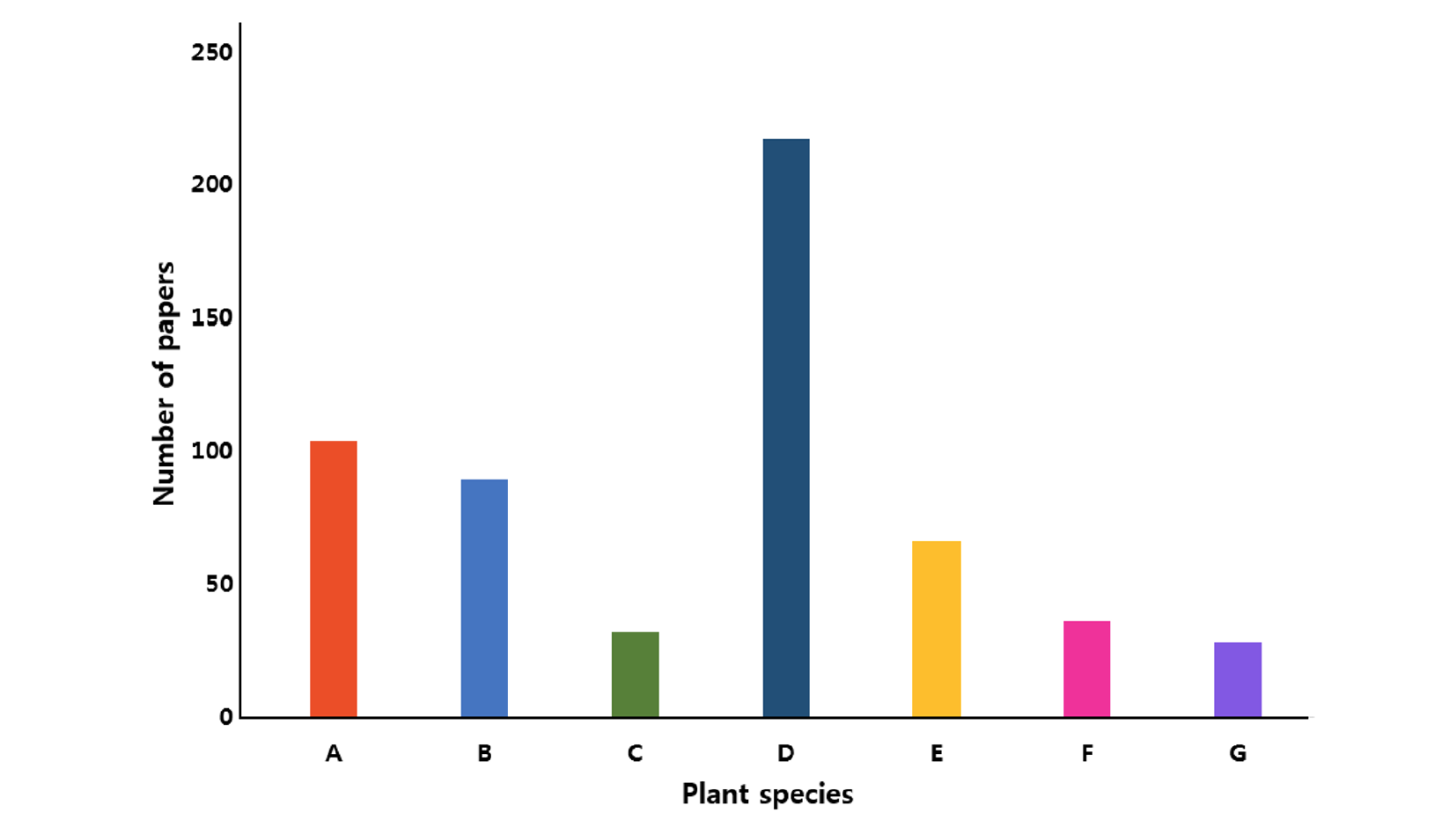
Fig. 7. Cumulative numbers of publications for plant species used for clustered regularly interspaced palindromic repeats (CRISPR)-mediated genome editing of various plant species. (A) Arabidopsis thaliana, (B) Solanum lycopersicum, (C) Solanum tuberosum, (D) Oryza sativa, (E) Triticum aestivum, (F) Glycine max, (G) Zea mays.
In general, ploidy of plants is another factor affecting the stability and efficiency of genome editing (Kishi-Kaboshi et al., 2017). As extra sets of chromosomes can manipulate cell size, color depth, and other features leading to morphological changes, polyploidy is common in ornamental cultivars (Manzoor et al., 2019). Complete knockout of multiple alleles of polyploid species becomes increasingly challenging as the number of chromosome sets increases (Yu et al., 2020). A few alleles may continue to function while simultaneous gene silencing is in process in transgenic plants, for which Agrobacterium has been used as the transformation mediator (Kishi-Kaboshi et al., 2017). Direct delivery of sgRNA and purified recombinant Cas9 by chemical and physical methods still faces the same dilemma (Andersson et al., 2017).
Selection of diploid cultivars as plant material may avoid the aforementioned difficulty in a limited manner; however, the use of experimental subjects with more than two sets of chromosomes is often inevitable because an ideal plant material would possess commercially favorable traits in addition to the gene of interest aimed for modification. Moreover, there are ample possibilities that genome-edited plant material is directly introduced to the horticulture industry; therefore, we suggest that researchers operate genome editing tools on competitive strains of plant material. As seen in the knock-out experiment of InCCD4 in Japanese morning glory (Watanabe et al., 2017), mutant lines showed a 20-fold increase in carotenoid accumulation relative to the wild type, but yellow coloration was not prominent owing to genetic limitations of the plant material used in the experiment. The results of the experiment implied the importance of the genetic capacity that the subject holds. Making use of haploid plants from pollen culture and microspore embryogenesis may increase the efficiency of site-directed mutagenesis in polyploid plant material (Wang et al., 2019a). The ploidy can be restored by chemical treatment such as the use of colchicine after evaluation of genome editing of haploid plant subjects (Saint-Oyant et al., 2018).
Tolerance of polyploidy and the mode of reproduction, wherein somatic mutations can be inherited through generations by differentiation of reproductive organs from multiple shoot meristems, enable plants to have higher mutation rates (Schoen and Schultz, 2019) in contrast to animals. Intra-specific and intra-cultivar variations in DNA can often be detected (Wang et al., 2019b), thus making it necessary for researchers to precisely sequence the target gene from their plant material. This issue results in more complicated operations to generate target-specific mutations in plant cells than in animals.
DNA-free genome editing
RNP in combination with gRNA and Cas9 can be directly introduced into competent protoplasts (Yu et al., 2020). Delivery of naked DNA material through the chemical action of PEG (Junker et al., 1987; Maas and Werr, 1989) and physical mechanisms of electroporation (Fromm et al., 1985; Fromm et al., 1987) is particularly important as introduced RNP molecules generate mutations similar to natural mutations in the target region and degenerate soon after the transfection, resulting in mutant protoplasts without any exogenous DNA material remaining. In addition, the two methods are most commonly used for transfection.
PEG-mediated transfection
PEG comprises repetitive ethylene glycol polymers. PEG is known to be innocuous to cells and is hydrophilic (Lee and Kim, 2005), making it an ideal reagent to help increase the solubility of proteins and genetic material by bioconjugation. Different PEGs with varying molecular weights are commercially available, and the broad range of use of PEG in biology is attributed to its low-cost, accessibility, and fair transfection efficiencies (Lee and Kim, 2005).
The conjugates from covalent binding of biomolecules with PEG have positive charges and interact with the negatively charged cell membranes of the protoplasts, resulting in uptake of the biomolecules into the cytoplasm through endocytosis (Wagner et al., 1991; Zalipsky and Harris, 1997). Woo et al. (2015) successfully introduced RNPs to protoplasts of Arabidopsis thaliana, tobacco, lettuce, and rice by PEG-mediated transfection in 2015, and this approach has also been adopted in petunia (Subburaj et al., 2016; Yu et al., 2020), apples, and grapes (Osakabe et al., 2018).
lectroporation
Electroporation is a process in which exogenous biomolecules are introduced to the cytoplasm through cell membranes that are temporarily permeabilized using electric pulses (Neuman, 1982). Cells in mixture with biomolecules are exposed to an intense electric field, and the lipid bilayer is disrupted to undergo electric breakdown. Increased conductance of cell membranes leads to transient pore formation, and external biomolecules can be transmitted to the cells (Neuman, 1982; Tsong, 1989; Tieleman, 2004; Potter and Heller, 2018). Although transfection by electroporation can be applied to any cell type, no published article in terms of delivery of RNP by electroporation in higher plants has been reported to date.
Future aspects of genome editing in ornamental species
Possession of attractive colors is one of the most important factors affecting customers’ purchase decisions in the floriculture industry (Behe et al., 1999). Chen et al. (2017) isolated an R2R3 transcription factor from grape hyacinth, namely MaAN2. Transformation and overexpression of MaAN2 in tobacco upregulated genes involved in anthocyanin biosynthesis and produced transgenic tobacco portraying visible dark coloration.
Vase life is another major factor that attracts customers (Rihn et al., 2014). Ethylene is a plant hormone associated with various developmental processes in plants (Burg and Burg, 1965). Elevated levels of exogenous and endogenous ethylene accelerate senescence by causing ethylene responses (Picton et al., 1993). Liu et al. (2016) silenced putative ethylene signaling downstream components EIL1 and EIL2 in petunia by the virus-induced gene silencing method. Their monitoring revealed that EIL genes are functionally redundant and down-regulation of the two genes influences the longevity of petunia flowers.
We expect that replacing one of the EIL genes (Solano et al., 1998; Liu et al., 2016) with MaAN2 (Chen et al., 2017) by CRISPR knock-in and enhancement of pigmentation by CRISPRa-facilitated upregulation of the transgene would simultaneously improve two categories of aesthetic quality in mutant lines: beauty and consistency of the beauty per se. The resulting plants would produce flowers with deep color and extended longevity, with negligible after-effects. Failure in ethylene perception often triggers severe maldevelopment in plants (Shibuya et al., 2004). However, silencing of an EIL gene did not display noticeable phenotypic changes other than extended flower longevity in transfected plants, indicating functional redundancy of EIL genes (Tieman et al., 2001; Liu et al., 2016).
Irrespective of the preference of patrons and the ease of detection, natural alteration of colors in plants is commonly observed (Sparrow and Sparrow, 1976). Because metabolic pathways related to the production of pigments incorporate diverse metabolites catalyzed by numerous enzymes, the chances of mutations are high in enzymes involved in complicated biosynthesis, such as anthocyanin and carotenoid synthesis (Clegg and Durbin, 2000; Tanaka et al., 2010). Flower phenotypes with pale colors or albinism often result from very small changes in base sequences (Clegg and Durbin, 2000; Nishihara et al., 2011) in the form of point mutations. The function of the mutated gene can be modified by CRISPR/Cas9 derived techniques and base editing, prime editing, and CRISPR knock-in through HDR are good candidates (Yu et al., 2020).
The emergence of the CRISPR/Cas system has replaced many existing genetic engineering techniques. Fresh approaches using the CRISPR system are being actively and continuously proposed and instantly applied, although validation of their safety, stability, and feasibility is ongoing. New advances and future discoveries of the system are expected to hasten plant breeding, and it will be up to society to assess its superior performance among other genome-editing tools.
Conclusion
The mainstream genome engineering community apparently favors the CRISPR/Cas system. In this review, we introduced several genome editing techniques derived from the CRISPR/Cas system within the scope of our knowledge and application of these tools in floricultural crops. We anticipate that our work will expand the reader’s imagination and understanding in terms of genome editing mediated by the CRISPR/Cas system. The development of genetic engineering has enabled mankind to decipher biological phenomena related to our well-being. The CRISPR/Cas system will pave the way toward new discoveries and applications much further than ever achieved through other technologies. We confidently assert that researchers working on breeding ornamental species will take advantage of the use of the CRISPR/Cas system.
Acknowledgements
This work was supported by a grant from the Plant Molecular Breeding Center of the Next Generation BioGreen21 Program (No. PJ01319303) and the New Breeding Technologies Development Program (No. PJ01485802), Rural Development Administration, Republic of Korea.
Authors Information
Hyunbae Lee, https://orcid.org/0000-0001-5512-5562
Saminathan Subburaj, https://orcid.org/0000-0002-9660-7661
Luhua Tu, https://orcid.org/0000-0003-1581-2282
Ka-Yeon Lee, https://orcid.org/0000-0003-2340-1846
Gwangsu Park, https://orcid.org/0000-0002-4668-2225
Geung-Joo Lee, https://orcid.org/0000-0002-3774-1860